
- Chinese Optics Letters
- Vol. 20, Issue 10, 100010 (2022)
Abstract
Keywords
1. Introduction
Organic-inorganic hybrid metal halide perovskites are a group of fast-developing materials for photovoltaic and optoelectronic applications[1–4]. Compared to their three-dimensional (3D) analogs, two-dimensional (2D) perovskites exhibit many advantages, including large exciton binding energy[5–7], improved moisture resistance[8–10], and the tunability of the bandgap by adjusting the layer thickness[11–13].
The biggest family of 2D perovskites is the Ruddlesden–Popper (R-P) phase. In their structure, two adjacent
We employ transient absorption (TA) and reflection (TR) experiments on the thin films of a 2D D-J phase perovskite,
Sign up for Chinese Optics Letters TOC. Get the latest issue of Chinese Optics Letters delivered right to you!Sign up now
Previously, bandgap oscillation induced by the optical excitation method has been observed in perovskites, which could be explained by local oscillation of lattices caused by optical phonons[17,18] or coherent interactions between light field and matter (the optical Stark effect)[19–21]. These are all short-time interactions, leading to fast bandgap oscillations on a picosecond scale. In this work, since the cause of oscillation is the longitudinal transport of acoustic phonons across the film, it displays a larger amplitude and a substantially longer period than previously reported.
2. Experiments
2D perovskite
Femtosecond TA/TR is based on a setup described previously[23]. Amplified 800 nm laser pulses are generated by a Coherent Astrella, which contains an oscillator and a Ti:sapphire regenerative amplifier. The output laser pulses have 1 kHz repetitive rate,
TA: Both pump and probe beams are incident on the sample from the perovskite–air interface. The probe beam transmits through the perovskite and substrate before collected by the detector. The probe incident angle is near normal, and the pump beam incident angle is slightly larger than that of the probe.
Front-face TR: The pump and probe are incident from the perovskite–air interface. The reflected probe from the perovskite–air interface is collected by the detector. The pump incident angle is near normal, and the probe incident angle is 45°.
Back-side transient reflection (BTR): The probe beams are incident on the sample from the perovskite–air interface, and the pump beam is shot on perovskite film from the substrate side. The reflected probe from the perovskite–air interface is collected by the detector. The pump incident angle is near normal, and the probe incident angle is 45°.
3. Results and Discussion
In this work, thin films of
Figure 1.Cross-sectional SEM images of the (DMAPA)PbI4 films.
TA measurement is carried out on a 286 nm thick film. The pump wavelength is at 400 nm (above bandgap), and the pumping energy density is at
Figure 2.(a) Scheme of TA experiments; (b) 2D contour plot of TA spectra; (c) ΔA spectra at selected time delays; (d) dependence of oscillation period and amplitude on film thickness; (e) dependence of oscillation period and amplitude on pump photon energy; (f) elucidating the origin of TA bandgap oscillation.
Previous reports show that bandgap oscillation observed in TA spectra of perovskites could be the result of many factors, such as coherent optical phonons and optical Stark effect. Laser pulses induce coherent lattice displacement and vibration, known as coherent optical phonons, and some vibrational modes will modulate the bandgap. The bandgap oscillation period is correlated to vibration frequency, which is on the order of femtoseconds to picoseconds[17]. The optical Stark effect is a coherent interaction between the light field itself and matter[24]. Therefore, the time scale of Stark-induced bandgap oscillation will not be longer than the bandwidth of laser pulses, which is tens to hundreds of femtoseconds. The period of bandgap oscillation in Fig. 1(a) is around tens to hundreds of picoseconds. It cannot be explained by optical phonons and the optical Stark effect.
To elucidate the bandgap oscillation mechanism observed in this work, we perform TA experiments on films with different thicknesses, using different pump wavelengths. The dependence of the oscillation on the pump wavelength and film thickness is shown in Figs. 2(d) and 2(e), respectively. Increasing the pump photon energy makes the oscillation amplitude stronger, indicating that the oscillation is induced by the excess energy of pump photon (2.53–3.10 eV) relative to
When the energy of the absorbed photon is higher than the bandgap, hot electron–hole pairs are generated. As hot excitons quickly cool down, excess energy is transferred to the perovskite lattice through electron–phonon coupling, generating a transient stress at the incident surface. This stress results in a strain wave (CLAP), which propagates from the surface into the deeper sample at the sound speed[25,26].
Figure 2(f) elucidates why the CLAP causes an oscillation in TA measurement. The CLAP contains a tensile strain at the wavefront and a compressive region behind; they are separated by the center of the CLAP[27]. The strain modifies the bandgap of the perovskite that it influences. The bleach peak is redshifted when the lattice is compressed, or blueshifted when the lattice is stretched[28]. As the CLAP bounces back and forth between the surfaces, the fraction of stretched/compressed regions alters. Considering that the TA is the averaged result of the photoinduced changes through the entire sample, a blueshift and a redshift of TA spectra are observed.
In the TA spectra, only one swing in bandgap is observed instead of periodic oscillations, indicating that the CLAP only bounces once on the substrate–perovskite interface. That is caused by the low reflectivity of the CLAP on the interface of perovskites and glass, which we used as the substrate. The reflection coefficient (
To verify the presence of the CLAP, a front-face TR experiment is performed using 400 nm pump beam. A 2D color map is shown in Fig. 3(b), where the color represents
Figure 3.(a) Scheme of front-face TR experiments; (b) 2D contour plot of TR spectra; (c) time-dependent ΔR/R at selected wavelengths; (d) dependence of oscillation period on wavelength of probe light; (e) elucidating the origin of oscillation observed in TR experiments.
In Fig. 3(b), an oscillation over time can also be observed. ΔR(t)/R(t) at different wavelengths are shown in Fig. 3(c). This figure shows that the oscillation periods at different wavelengths are different. The periods are calculated by a fast Fourier transform (FFT) and plotted in Fig. 3(d). The oscillation period was positively correlated with the probe wavelength, confirming the cross-plane ballistic transportation of CLAPs[31].
Figure 3(e) explains this time-dependent oscillation. The CLAP itself is a discontinuity of the refractive index; therefore it can reflect the probe beam. The probe light reflected by the CLAP interferes with those reflected at the perovskite–air interface. As the CLAP is propagating into the sample at sound velocity, the interfering distance is changed, leading to the oscillation in probe intensity. The oscillation period
To gain a deeper insight into the influence of the CLAP, BTR measurement is performed under a 400 nm pump. Figure 4(b) shows the 2D contour plot of BTR spectra of a 704 nm-thick
Sign up for Chinese Optics Letters TOC. Get the latest issue of Chinese Optics Letters delivered right to you!Sign up now
Figure 4.(a) Scheme of BTR experiments; (b) 2D contour plot of BTR spectra; (c) time-dependent ΔR/R at selected wavelengths; (d) time when the CLAP arrives at air–perovskite interfaces for films of different thicknesses; (e) elucidating the origin of oscillation, and the CLAP arrival time observed in BTR experiments.
In Fig. 4(c), which plots the time evolution of
The arrival time should be correlated to film thickness, so we performed BTR measurement on samples with different thicknesses. The CLAP arrival time in films at different thicknesses is shown in Fig. 4(d). A linear correlation is displayed, indicating that the CLAP transports ballistically inside the perovskite film. The slope of the curve, 2386 m/s, is the sound velocity in the film. This number is larger than the sound velocity in typical 2D R-P perovskites[32], indicating more efficient transport of acoustic phonons in the lattice of the D-J phase.
We also attempt to look for CLAPs in 3D (
4. Conclusions
In this work, we observe a strong and slow bandgap oscillation in the thin film of a 2D D-J phase perovskite,
The oscillation has a dependence on the pump photon energy and film thickness, suggesting it originates from the transport of CLAPs across the film. When the energy in the excitation photon is larger than perovskite bandgap, excess energy is transferred into the lattice, generating acoustic phonons and a local strain. The strain propagates in the film by the speed of sound, modulating the bandgap of the lattice around it. As the CLAP moves, the ratio between compressed and stretched regions changes, making the spectra oscillate.
The presence of the CLAP is confirmed by the TR experiments. In front-face TR, spectral oscillation is also observed. This oscillation is caused by the interference of the probe laser beam reflected at the air–perovskite interface and reflected by the CLAP. The motion of the CLAP changes the interference distance, leading to the spectral oscillation. In BTR, the arrival of the CLAP at the free surface was observed, and the sound velocity was calculated to be 2386 m/s with that information.
In 3D and 2D R-P phase perovskites, notable CLAP transport can only be observed in TR experiments. Large bandgap oscillation perovskites from the TA experiments indicate a stronger coupling between carriers and lattice and the larger effect of local strain on band energy in the D-J phase. This observation provides opportunities for future dynamical bandgap modulation in D-J phase perovskites.
References
[1] D. Li, D. Zhang, K. S. Lim, Y. Hu, Y. Rong, A. Mei, N. G. Park, H. Han. A review on scaling up perovskite solar cells. Adv. Funct. Mater., 31, 2008621(2020).
[2] R. Azmi, E. Ugur, A. Seitkhan, F. Aljamaan, A. S. Subbiah, J. Liu, G. T. Harrison, M. I. Nugraha, M. K. Eswaran, M. J. S. Babics. Damp heat–stable perovskite solar cells with tailored-dimensionality 2D/3D heterojunctions. Science, 376, 73(2022).
[3] H. Zhu, Y. Ren, L. Pan, O. Ouellette, F. T. Eickemeyer, Y. Wu, X. Li, S. Wang, H. Liu, X. Dong, S. M. Zakeeruddin, Y. Liu, A. Hagfeldt, M. Gratzel. Synergistic effect of fluorinated passivator and hole transport dopant enables stable perovskite solar cells with an efficiency near 24. J. Am. Chem. Soc., 143, 3231(2021).
[4] Y. Yin, W. Tian, H. Luo, Y. Gao, T. Zhao, C. Zhao, J. Leng, Q. Sun, J. Tang, P. Wang, Q. Li, X. Lü, J. Bian, S. Jin. Excellent carrier transport property of hybrid perovskites sustained under high pressures. ACS Energy Lett., 7, 154(2021).
[5] L. N. Quan, M. Yuan, R. Comin, O. Voznyy, E. M. Beauregard, S. Hoogland, A. Buin, A. R. Kirmani, K. Zhao, A. Amassian, D. H. Kim, E. H. Sargent. Ligand-stabilized reduced-dimensionality perovskites. J. Am. Chem. Soc., 138, 2649(2016).
[6] Q. Sun, C. Zhao, Z. Yin, S. Wang, J. Leng, W. Tian, S. Jin. Ultrafast and high-yield polaronic exciton dissociation in two-dimensional perovskites. J. Am. Chem. Soc., 143, 19128(2021).
[7] W. Tao, Y. Zhang, H. Zhu. Dynamic exciton polaron in two-dimensional lead halide perovskites and implications for optoelectronic applications. Acc. Chem. Res., 55, 345(2022).
[8] G. Wu, R. Liang, M. Ge, G. Sun, Y. Zhang, G. Xing. Surface passivation using 2D perovskites toward efficient and stable perovskite solar cells. Adv. Mater., 34, 2105635(2022).
[9] X. Zhao, T. Liu, Y. L. Loo. Advancing 2D perovskites for efficient and stable solar cells: challenges and opportunities. Adv. Mater., 34, 2105849(2022).
[10] J. Tang, W. Tian, C. Zhao, Q. Sun, C. Zhang, H. Cheng, Y. Shi, S. Jin. Imaging the moisture-induced degradation process of 2D organolead halide perovskites. ACS Omega, 7, 10365(2022).
[11] C. Katan, N. Mercier, J. Even. Quantum and dielectric confinement effects in lower-dimensional hybrid perovskite semiconductors. Chem. Rev., 119, 3140(2019).
[12] C. M. Mauck, W. A. Tisdale. Excitons in 2D organic–inorganic halide perovskites. Trends Chem., 1, 380(2019).
[13] J. C. Blancon, J. Even, C. C. Stoumpos, M. G. Kanatzidis, A. D. Mohite. Semiconductor physics of organic-inorganic 2D halide perovskites. Nat. Nanotechnol., 15, 969(2020).
[14] L. Mao, W. Ke, L. Pedesseau, Y. Wu, C. Katan, J. Even, M. R. Wasielewski, C. C. Stoumpos, M. G. Kanatzidis. Hybrid Dion-Jacobson 2D lead iodide perovskites. J. Am. Chem. Soc., 140, 3775(2018).
[15] P. Fu, Y. Liu, S. Yu, H. Yin, B. Yang, S. Ahmad, X. Guo, C. Li. Dion-Jacobson and Ruddlesden-Popper double-phase 2D perovskites for solar cells. Nano Energy, 88, 106249(2021).
[16] J. Gong, M. Hao, Y. Zhang, M. Liu, Y. Zhou. Layered 2D halide perovskites beyond the Ruddlesden–Popper phase: tailored interlayer chemistries for high-performance solar cells. Angew. Chem. Int. Ed., 61, e202112022(2022).
[17] P. Guo, Y. Xia, J. Gong, D. H. Cao, X. Li, X. Li, Q. Zhang, C. C. Stoumpos, M. S. Kirschner, H. Wen, V. B. Prakapenka, J. B. Ketterson, A. B. F. Martinson, T. Xu, M. G. Kanatzidis, M. K. Y. Chan, R. D. Schaller. Direct observation of band gap oscillations induced by optical phonons in hybrid lead iodide perovskites. Adv. Funct. Mater., 30, 1907982(2020).
[18] H. Kim, J. Hunger, E. Canovas, M. Karakus, Z. Mics, M. Grechko, D. Turchinovich, S. H. Parekh, M. Bonn. Direct observation of mode-specific phonon-band gap coupling in methylammonium lead halide perovskites. Nat. Commun., 8, 687(2017).
[19] Y. Li, S. He, X. Luo, X. Lu, K. Wu. Strong spin-selective optical Stark effect in lead halide perovskite quantum dots. J. Phys. Chem. Lett., 11, 3594(2020).
[20] Y. Yang, M. Yang, K. Zhu, J. C. Johnson, J. J. Berry, J. van de Lagemaat, M. C. Beard. Large polarization-dependent exciton optical Stark effect in lead iodide perovskites. Nat. Commun., 7, 12613(2016).
[21] D. Giovanni, W. K. Chong, H. A. Dewi, K. Thirumal, I. Neogi, R. Ramesh, S. Mhaisalkar, N. Mathews, T. C. Sum. Tunable room-temperature spin-selective optical Stark effect in solution-processed layered halide perovskites. Sci. Adv., 2, e1600477(2016).
[22] W. Zhao, Q. Dong, J. Zhang, S. Wang, M. Chen, C. Zhao, M. Hu, S. Jin, N. P. Padture, Y. Shi. Asymmetric alkyl diamine based Dion–Jacobson low-dimensional perovskite solar cells with efficiency exceeding 15%. J. Mater. Chem. A, 8, 9919(2020).
[23] J. Liu, J. Leng, K. Wu, J. Zhang, S. Jin. Observation of internal photoinduced electron and hole separation in hybrid two-dimensional perovskite films. J. Am. Chem. Soc., 139, 1432(2017).
[24] S. H. Autler, C. H. Townes. Stark effect in rapidly varying fields. Phys. Rev., 100, 703(1955).
[25] C. Thomsen, J. Strait, Z. Vardeny, H. J. Maris, J. Tauc, J. J. Hauser. Coherent phonon generation and detection by picosecond light pulses. Phys. Rev. Lett., 53, 989(1984).
[26] P. Ruello, V. E. Gusev. Physical mechanisms of coherent acoustic phonons generation by ultrafast laser action. Ultrasonics, 56, 21(2015).
[27] C. Thomsen, H. T. Grahn, H. J. Maris, J. Tauc. Surface generation and detection of phonons by picosecond light pulses. Phys. Rev. B, 34, 4129(1986).
[28] D. Liu, D. Luo, A. N. Iqbal, K. W. P. Orr, T. A. S. Doherty, Z. H. Lu, S. D. Stranks, W. Zhang. Strain analysis and engineering in halide perovskite photovoltaics. Nat. Mater., 20, 1337(2021).
[29] E. T. Swartz, R. O. Pohl. Thermal boundary resistance. Rev. Mod. Phys., 61, 605(1989).
[30] Y. Yang, M. Yang, D. T. Moore, Y. Yan, E. M. Miller, K. Zhu, M. C. Beard. Top and bottom surfaces limit carrier lifetime in lead iodide perovskite films. Nat. Energy, 2, 16207(2017).
[31] J. K. Miller, J. Qi, Y. Xu, Y. J. Cho, X. Liu, J. K. Furdyna, I. Perakis, T. V. Shahbazyan, N. Tolk. Near-band gap wavelength dependence of long-lived traveling coherent longitudinal acoustic phonons in GaSb-GaAs heterostructures. Phys. Rev. B, 74, 113313(2006).
[32] P. Guo, C. C. Stoumpos, L. Mao, S. Sadasivam, J. B. Ketterson, P. Darancet, M. G. Kanatzidis, R. D. Schaller. Cross-plane coherent acoustic phonons in two-dimensional organic-inorganic hybrid perovskites. Nat. Commun., 9, 2019(2018).
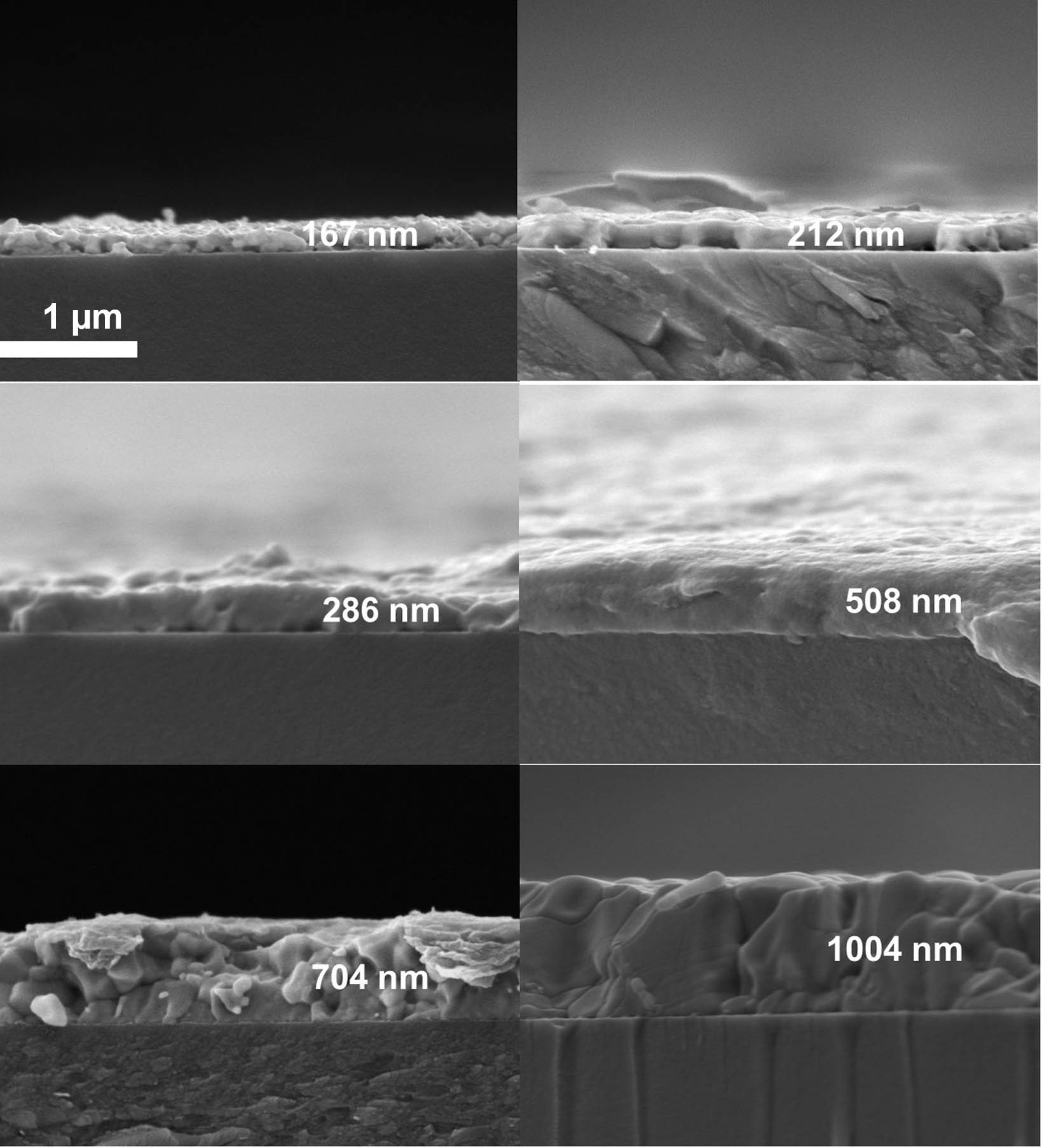
Set citation alerts for the article
Please enter your email address