
- Photonics Research
- Vol. 10, Issue 2, 381 (2022)
Abstract
1. INTRODUCTION
The transformation of information storage has brought people into the rapidly growing “era of digital information explosion,” which has stimulated updating secure storage media and various storage methods [1]. At present, optical data storage has become the new generation of information storage stars because of its advantages of high efficiency, energy conservation, environmental protection, privacy, repeatable writing, and reading [2–6].
Recently, a long persistent luminescence (LPL) medium with excellent performance has emerged and shines brightly in the field of optical data storage. Zhuang reported, for example, that spherical
Since Matsuzawa’s discovery of green
Sign up for Photonics Research TOC. Get the latest issue of Photonics Research delivered right to you!Sign up now
Herein, we develop a glass ceramic via
2. EXPERIMENTAL SECTION
An STA 449F30020 synchronous thermal analyzer was used to obtain the differential scanning curve (DSC). The crystallite phase of glass samples was identified via X-ray diffraction (XRD) measurement (D2 ADVANCE/Germany Bruker phaser diffractometer) with
3. RESULTS AND DISCUSSION
Figure 1.Crystallization of glass samples for
Besides, Figs. 1(h
The B25 and B30 samples growing
Figure 2.PL properties of
Through trapping of the TRL curve in Fig. 3(a), analysis reveals the LPL mechanism in the
Figure 3.LPL property and mechanism in the
The proposed energy-level diagram for explaining the charge carrier transition process in
Figure 4 explores the potential application of glass in the field of information storage. A special photomask is irradiated by UV light, and the information of the photomask is stored in the glass in the form of photons. The optical information was engraved into the glass after turning off the UV light source, which is unrecognizable after being placed in a dark room for 30 h. The information can be read out again in the following three methods. (i) Place the glass in 100°C water or (ii) place the glass in a furnace at about 440 K. Both methods are to release the electrons of the deep trap through thermal stimulation. (iii) Release the electrons of the deep trap through optical stimulation under 980 nm light irradiation (surface scanning irradiation). Finally, this optical information can be quickly eliminated without a long wait, i.e., placing the glass in a furnace higher than 663 K, which will completely release the electrons in the deep trap and return the sample to its original state.
Figure 4.Glass pictures of writing, reading, and erasing processes of optical information.
4. CONCLUSION
In summary,
Acknowledgment
Acknowledgment. We thank Yongmin Duan and Yang Lu for providing constructive criticism for this paper.
References
[1] M. Gu, X. Li, Y. Cao. Optical storage arrays: a perspective for future big data storage. Light Sci. Appl., 3, e177(2014).
[2] W. Zhang, X. Liu, Q. Li, H. Tang, J. Xie, Z. Wang, C. Wang, L. Jiang, X. Zhang. Excellent thermal stability of Y2.94Al4−
[3] X. Lin, K. Deng, H. Wu, B. Du, B. Viana, Y. Li, Y. Hu. Photon energy conversion and management in SrAl12O19: Mn2+, Gd3+ for rewritable optical information storage. Chem. Eng. J., 420, 129844(2021).
[4] Z. Long, J. Zhou, J. Qiu, Q. Wang, Y. Li, J. Wang, D. Zhou, Y. Yang, H. Wu, Y. Wen. Thermal engineering of electron-trapping materials for “Smart-Write-In” optical data storage. Chem. Eng. J., 420, 129788(2021).
[5] P. Wen, Y. Xu, S. Li, Z. Sun, M. Panmai, J. Xiang, S. Tie, S. Lan. Two-dimensional closely-packed gold nanoislands: a platform for optical data storage and carbon dot generation. Appl. Surf. Sci., 555, 149586(2021).
[6] Z. Hu, X. Huang, Z. Yang, J. Qiu, Z. Song, J. Zhang, G. Dong. Reversible 3D optical data storage and information encryption in photo-modulated transparent glass medium. Light Sci. Appl., 10, 140(2021).
[7] Y. Zhuang, D. Chen, W. Chen, W. Zhang, X. Su, R. Deng, Z. An, H. Chen, R. J. Xie. X-ray-charged bright persistent luminescence in NaYF4:Ln3+@NaYF4 nanoparticles for multidimensional optical information storage. Light Sci. Appl., 10, 132(2021).
[8] Y. Wang, D. Chen, Y. Zhuang, W. Chen, H. Long, H. Chen, R. J. Xie. NaMgF3:Tb3+@NaMgF3 nanoparticles containing deep traps for optical information storage. Adv. Opt. Mater., 9, 2100624(2021).
[9] S. Lin, H. Lin, C. Ma, Y. Cheng, S. Ye, F. Lin, R. Li, J. Xu, Y. Wang. High-security-level multi-dimensional optical storage medium: nanostructured glass embedded with LiGa5O8:Mn2+ with photostimulated luminescence. Light Sci. Appl., 9, 22(2020).
[10] T. Matsuzawa, Y. Aoki, N. Takeuchi, Y. Murayama. A new long phosphorescent phosphor with high brightness, SrAl2O4:Eu2+, Dy3 +. J. Electrochem. Soc., 143, 2670-2673(2019).
[11] Z. Xue, S. Deng, Y. Liu. Synthesis and luminescence properties of SrAl2O4:Eu2+, Dy3+ nanosheets. Physica B, 407, 3808-3812(2012).
[12] Y. F. Xu, D. K. Ma, M. L. Guan, X. A. Chen, Q. Q. Pan, S. M. Huang. Controlled synthesis of single-crystal SrAl2O4:Eu2+, Dy3+ nanosheets with long-lasting phosphorescence. J. Alloys Compd., 502, 38-42(2010).
[13] H. Song, D. Chen. Combustion synthesis and luminescence properties of SrAl2O4:Eu2+, Dy3+, Tb3+ phosphor. Luminescence, 22, 554-558(2007).
[14] S. Yu, P. Pi, X. Wen, J. Cheng, Z. Yang. Preparation and luminescence of SrAl2O4:Eu2+, Dy3+ phosphors coated with maleic anhydride. Can. J. Chem. Eng., 86, 30-34(2008).
[15] J. M. Ngaruiya, S. Nieuwoudt, O. M. Ntwaeaborwa, J. J. Terblans, H. C. Swart. Resolution of Eu2+ asymmetrical emission peak of SrAl2O4:Eu2+, Dy3+ phosphor by cathodoluminescence measurements. Mater. Lett., 62, 3192-3194(2008).
[16] P. Roldán Del Cerro, T. Salminen, M. Lastusaari, L. Petit. Persistent luminescent borosilicate glasses using direct particles doping method. Scr. Mater., 151, 38-41(2018).
[17] T. Nakanishi. Preparation of europium-activated SrAl2O4 glass composites using the frozen sorbet technique. J. Ceram. Soc. Jpn., 123, 862-867(2015).
[18] K. Shinozaki, T. Honma, M. Affatigato, T. Komatsu. Long afterglow in hexagonal SrAl2O4:Eu2+, Dy3+ synthesized by crystallization of glass and solidification of supercooled melts. J. Lumin., 177, 286-289(2016).
[19] M. Yamashita, T. Imamura, S. Matsumoto, M. Murakami, T. Hongo, T. Akai, Y. Iwamoto. Enhancement of afterglow luminescence of long-lasting phosphor-glass composite by using refractive index matched glass. Key Eng. Mater., 702, 113-117(2016).
[20] H. Yoshida, S. Fujino, T. Kajiwara. Afterglow luminance property of phosphorescent phosphor SrAl2O4:Eu2+, Dy3+-glass composites. J. Ceram. Soc. Jpn., 118, 784-787(2010).
[21] B. Cheng, Z. Zhang, Z. Han, Y. Xiao, S. Lei. SrAl2O4:Eu2+, Dy3+ nanobelts: synthesis by combustion and properties of long-persistent phosphorescence. J. Mater. Res. Technol., 26, 2311-2315(2011).
[22] Y. Wu, J. Gan, X. Wu. Study on the silica-polymer hybrid coated SrAl2O4:Eu2+, Dy3+ phosphor as a photoluminescence pigment in a waterborne UV acrylic coating. J. Mater. Res. Technol., 13, 1230-1242(2021).
[23] T. Cai, S. Guo, Y. Li, D. Peng, X. Zhao, W. Wang, Y. Liu. “Ultra-sensitive mechanoluminescent ceramic sensor based on air-plasma-sprayed SrAl2O4:Eu2+, Dy3+ coating. Sens. Actuators A, 315, 112246(2020).
[24] T. Nakanishi, Y. Katayama, J. Ueda, T. Honma, S. Tanabe, T. Komatsu. Fabrication of Eu:SrAl2O4-based glass ceramics using frozen sorbet method. J. Ceram. Soc. Jpn., 119, 609-615(2011).
[25] X. Xu, Y. Wang, C. Zu, P. Zhou. Effect of 3B group in rings on special dispersion of B2O3-SiO2-ZrO2-Ta2O5-Na2O system glass. J. Wuhan Univ. Technol., 33, 1032-1038(2018).
[26] K. Ding, N. Chen, G. Du, A. Zhang. Preparation, structures, thermal properties and sintering behaviors of B2O3-SiO2-ZnO-BaO-Al2O3 glass. J. Wuhan Univ. Technol., 31, 1323-1328(2016).
[27] Y. Duan, P. Li, Y. Lu, S. Xu, J. Zhang. Enhanced luminescence of self-crystallized Cs4PbBr6 quantum dots via regulating glass ceramic network structure. Ceram. Int., 47, 24198-24206(2021).
[28] J. Cui, X. Cao, L. Shi, Z. Zhong, P. Wang, L. Ma. The effect of substitution of Al2O3 and B2O3 for SiO2 on the properties of cover glass for liquid crystal display: structure, thermal, visco-elastic, and physical properties. Int. J. Appl. Glass Sci., 12, 443-456(2021).
[29] O. Sherstyuk, A. Ivanova, M. Lebedev, M. Bukhtiyarova, L. Matvienko, A. Budneva, A. Simonov, V. Yakovlev. Transesterification of rapeseed oil under flow conditions catalyzed by basic solids: M-Al(La)-O (M = Sr, Ba), M-Mg-O (M = Y, La). Appl. Catal. A, 419-420, 73-83(2012).
[30] J. Xu, Z. Chen, M. Gai, Y. Fan, C. He. Optically stimulated luminescence of Dy3+-doped NaCaPO4 glass-ceramics. J. Rare Earths, 38, 927-932(2020).
[31] C. Wang, Y. Jin, Y. Lv, G. Ju, D. Liu, L. Chen, Z. Li, Y. Hu. Trap distribution tailoring guided design of super-long-persistent phosphor Ba2SiO4:Eu2+, Ho3+ and photostimulable luminescence for optical information storage. J. Mater. Chem. C, 6, 6058-6067(2018).
[32] R. Chen. On the calculation of activation energies and frequency factors from glow curves. J. Appl. Phys., 40, 570-585(1969).
[33] Y. Jia, W. Sun, R. Pang, T. Ma, D. Li, H. Li, S. Zhang, J. Fu, L. Jiang, C. Li. Sunlight activated new long persistent luminescence phosphor BaSiO3:Eu2+, Nd3+, Tm3+: optical properties and mechanism. Mater. Des., 90, 218-224(2016).
[34] W. Wang, J. Yang, Z. Zou, J. Zhang, H. Li, Y. Wang. An isolated deep-trap phosphor for optical data storage. Ceram. Int., 44, 10010-10014(2018).
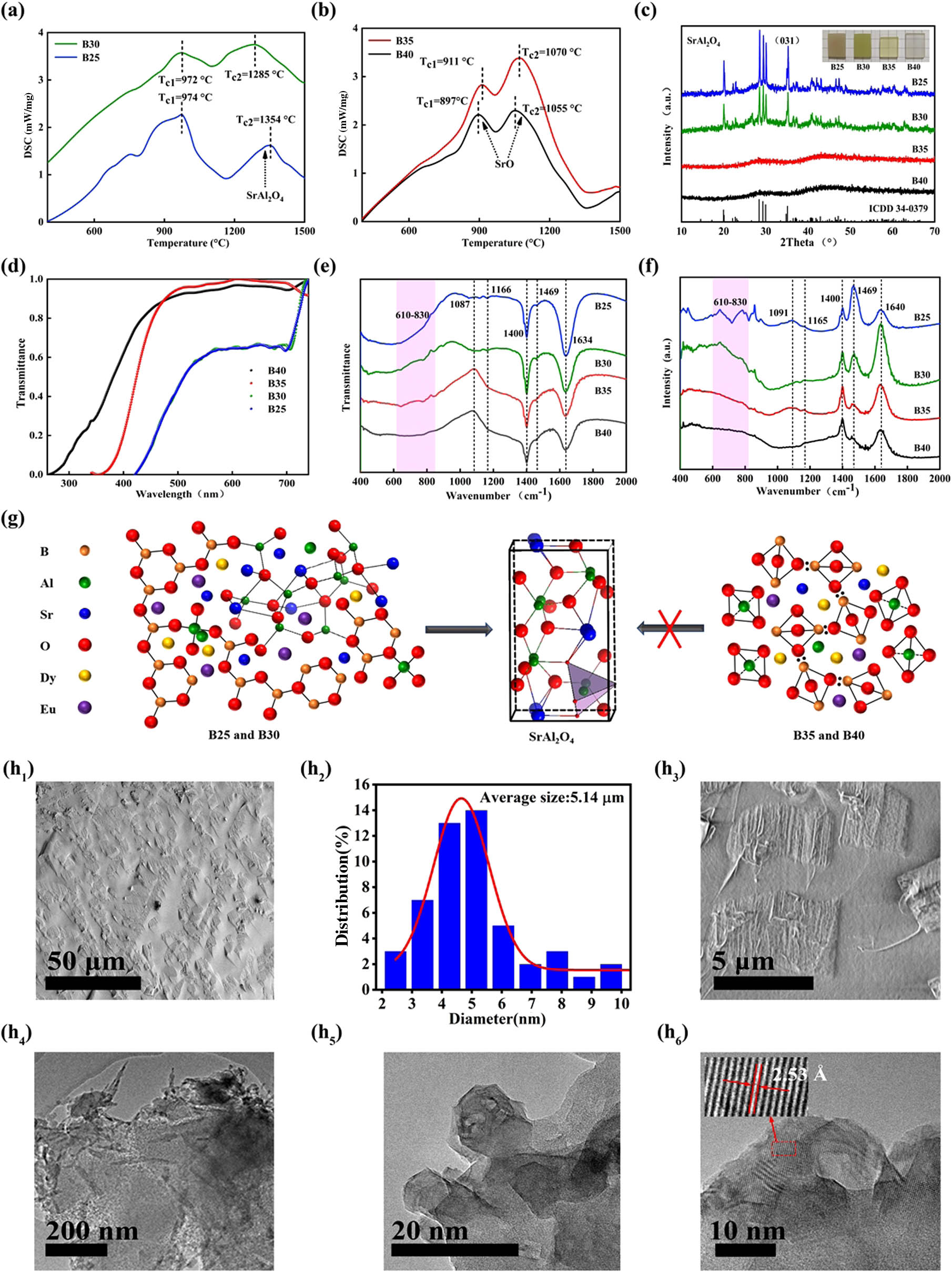
Set citation alerts for the article
Please enter your email address