Abstract
A novel black phosphorus (BP) solution saturable absorber (SA) is fabricated by the liquid-phase-exfoliated method and successfully used for passively -switched (QS) laser. Compared with a traditional solid SA, a BP solution SA possesses more excellent optical transparency and higher damage resistance. The shortest pulse duration and highest average output power are measured to be 119 ns and 1.23 W, respectively. To the best of our knowledge, both of them are the best results among QS solid-state lasers with BP-based absorbers so far. The repetition rate is in the range of 533.2 to 722 kHz. The results indicate the potential application of the BP solution SA into high-power solid-state pulse lasers.High power -switched (QS) laser pulses of nanosecond time duration always have extensive applications in the fields of material processing, biomedicine, spectroscopy, and so on. Therefore, the manufacture of QS solid-state lasers (SSLs) with high output power and narrow pulse width is extremely essential. As a significant technique for generating nanosecond laser pulses, passively -switching in SSLs depends absolutely on high-quality saturable absorbers (SAs)[1,2]. Since 2009, due to the intrinsic zero bandgap and large nonlinear absorption wavelength range[3–5], Graphene has been extensively applied to the pulse generation of various wave bands[3–7]. The successful applications of graphene inspire researchers to explore other graphene-like two-dimensional (2D) materials such as topological isolators (TIs)[8,9] and transition metal dichalcogenides (TMDs)[10,11]. Over the last few years, these emerging 2D materials have experienced a rapid development. Many kinds of SAs fabricated with 2D materials are used for mode locking or -switching technology in different lasers[6–22]. Recently, as another kind of 2D nanomaterial, black phosphorus (BP) has attracted significant attention in the field of nonlinear optical materials. Compared with other allotropes of phosphorus, BP possesses a “wrinkled” layered structure and a relatively strong thermodynamic stability[23,24]. In addition, BP is a high-mobility semiconductor with a direct bandgap sensitivity depending on the number of layers from 0.3 (bulk) to 2.0 eV (single layer) that exactly fill the gap between the zero bandgap of graphene and the wide bandgap (1.0–2.0 eV) of TMDs[25]. The narrower bandgap of BP makes itself act as the SA, which has been used in a broader wavelength operation range of 4–0.8 μm[26,27]. In addition, like graphene, BP SA owns the same excellent nonlinear optical response and easy fabrication process[13,23].
-switching or mode locking in SSLs using graphene-like absorbers[9,15,16,18,20,21,26,27,29–35] are listed in Table 1. These absorbers are made by growing nanomaterials by chemical vapor deposition and transferring themon a mirror or depositing them on the quartz substrate by the spin-coating method. However, even if the reports are numerous, the average output power of the pulsed lasers is very limited. The maximum average output power reported is only 820 mW[33] (-switching)[29] (mode locking). The low output power is mainly attributed to the low laser damage threshold of the 2D material. Although several mode locking operations[20,21,29,32] are reported, most of the Letters are about QS lasers. The shortest pulse width of the reported Letters is 97 ns with a absorber[34]. For BP absorbers, QS pulses with widths of 189[26], 495[30], and 636 ns[26] have been generated corresponding, respectively, to the wavelengths of 0.6, 1, and 2 μm.
SA | Gain medium | Wavelengh (nm) | Laser operation | Pulse width | Output power (mW) | Reference |
---|
Bi2Se3 SAM | Nd:YVO4 | 1066 | QS | 250 ns | 74.2 | [9] |
Bi2Se3 quartz substrate | Nd:GdVO4 | 1063 | QS | 666 ns | 32 | [35] |
Bi2Te3 | Nd:YVO4 | 1064 | QS | 97 ns | 18.6 | [34] |
Bi2Se3 thin film | Yb:KGW | 1042 | QS | 1.5 μs | 820 | [33] |
MoS2-BK7 | Nd:YAlO3 | 1079 | QS | 227 ns | 260 | [15] |
MoS2 mica | Tm:GdVO4 | 1902 | QS | 800 ns | 100 | [16] |
MoS2 substrate | Yb:LGGG | 1025–1028 | QS | 182 ns | 600 | [18] |
WS2SiO2 substrate | Yb:YAG | 1050 | ML | 736 fs | 270 | [20] |
MoS2 SAM | Nd:YVO4 | 1064 | ML | 12.7 ps | 89 | [21] |
BP SAM | Yb,Lu:GALGO | 1053 | ML | 272 fs | 820 | [29] |
BP quartz glass | Yb:ScBO3 | 1063 | QS | 495 ns | 167 | [30 |
BP SAM | Cr:ZnSe | 2400 | QS | 189 ns | 36 | [31] |
BP quartz glass | Pr:GdLiF4Nd:GdVO4 Tm:Ho:YGG | 639 1064 2100 | QS | 189 ns 495 ns 636 ns | 18 22 27 | [26] |
BP gold-film mirror | Yb:LuYAG Tm:CaYAlO4Er:Y2O3 | 1030 1930 2720 | QS | 1.73 μs 3.1 μs 4.47 μs | 6 12 6 | [27] |
BP quartz plate | Nd:YVO4 | 1064 | ML | 6.1 ps | 460 | [32] |
BP solution SA | Nd:YVO4 | 1064 | QS | 119 ns | 1230 | Our work |
Table 1. Pulsed SSLs Based on Graphene-like 2D Materials
In this Letter, a BP solution absorber is fabricated and successfully employed in a QS laser oscillator. The solvent of the SA can dissipate heat from the surfaces of BP nanosheets rapidly, which increases the laser damage resistance of the absorber and helps it withstand high laser power illumination. By inserting a BP solution SA into an laser cavity, we obtain the pulses with a duration of 119 ns and an average output power of 1.23 W. To the best of our knowledge, the pulse duration is the shortest and the average output power is the highest among the QS SSLs with BP-based absorbers so far. Furthermore, it is the first time that watt-level pulsed lasers based on emerging graphene-like 2D nanomaterials are presented.
Sign up for Chinese Optics Letters TOC. Get the latest issue of Chinese Optics Letters delivered right to you!Sign up now
In order to fabricate high-quality BP SAs, the BP nanosheets are exfoliated by the liquid-phase-exfoliation method[13]. First, four equivalent amounts of bulk BP powders are dispersed, respectively, in four equivalent volume prepared solvents [, ethanol, isopropyl alcohol (IPA), and n-methylpyrrolidone (NMP)] and followed by high power ultrasonic oscillation for 5 h. To remove the residual bulk BP, the dispersions are centrifuged at 6000 rpm for 6 min, and the supernatant solutions are taken for standby. Finally, we get four bottles of stable BP solutions, as shown in Fig. 1(a). From the figure we can see that the BP-IPA solution and the BP-NMP solution present a flavescent color compared with the almost transparent and BP-ethanol, which illustrates that BP nanosheets in organic solvents of IPA and NMP have a high solubility. Therefore, IPA and NMP are suitable as solvents of the BP solution SA. To pick out the best candidate, we measure the transmission of , ethanol, IPA, NMP, BP-IPA, and BP-NMP samples with an optical spectrometer, scanning from 800 to 1100 nm. Six kinds of solutions are filled in the same quartz cell (2 mm thickness) successively and put in the sample pool vertically. The transmission curves are depicted in Fig. 1(b). It can be clearly observed that IPA and NMP solvents have a better optical transparency than that of or ethanol, and the transmission of NMP is relatively higher than that of IPA in the spectral region from 950 to 1100 nm. The absorption of BP nanosheets dissolved in NMP is 1.7%, which is stronger than that in IPA (about 0.7%) at the wavelength of 1064 nm. Under the same preparation conditions, a higher absorption represents a higher concentration of BP nanosheets remaining in the solvent, which is helpful to generate pulses with a narrow duration[22]. Ultimately, we choose the BP-NMP solution as the SA for -switching operation. To measure the Raman spectrum of BP nanosheets, the BP-NMP solution is dripped on a 1 mm thickness quartz plate and then dried under an oven lamp. We repeat the steps until the quartz is covered with BP nanosheets. The Raman spectrum of the as-prepared BP sample is measured by a Raman spectrometer excited by a 532 nm laser source, as displayed in Fig. 1(c). We observe that three peaks appear at , , and , corresponding to (one out-of-plane vibration mode of phosphorus atoms), , and (two in-plane oscillations of phosphorus atoms in the BP layer), respectively. The positions of three Raman peaks are nearly consistent with the previously reported results[23,25–27]. Figure 1(d) shows the nonlinear transmission curve of the BP solution SA, which is measured by a 150 fs titanium gem laser at 800 nm with a repetition rate of 50 kHz. The experimental results show a typical saturable absorption and can be well fitted by the nonlinear saturable absorption equation. The modulation depth and saturation intensity of our BP SA is about 6.3% and , respectively.
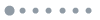
Figure 1.(a) BP nanosheets solution. (b) Transmission spectra. (c) Raman spectra of BP. (d) The nonlinear transmission curve of BP solution SA.
Figure 2 shows the schematic of a passively QS laser with an 18 cm long V-type cavity (, , ). A a-cut crystal with 0.7 at. % is employed as the gain media, and two light-passing faces of the crystal are coated for 808 nm and 1064 nm antireflection (AR) film. In order to improve the thermal diffusion, the crystal is wrapped with indium foil and mounted in a water-cooled copper block maintained at 21°C by a cool-water machine[27,35]. An 808 nm fiber-coupled diode laser with a core diameter of 200 μm and a numerical aperture of 0.22 is used as the pump source, of which the maximum output power is 30 W. Through a optical focusing system, the pump laser is focused on the gain crystal with a diameter of 400 μm. The end mirror is a dichroic mirror coated for 808 nm AR and 1064 nm high-reflection (HR) film. The plano-concave mirror with a radius of curvature of 100 mm has a transmission of 15% at the laser wavelength of 1064 nm so that it can be not only used as folding mirrors to conduct the spot size on the surface of SA but also act as an output coupler (OC). There are two laser beams that transmit through it because the OC is not an end mirror of the cavity. The BP-NMP solution SA is filled into a 10 mm thickness quartz cell. One of the outside faces of the quartz cell is coated with 1064 nm HR film and the other outside face of the quartz cell is coated with 1064 nm AR film so that the cell acts as a reflection-type absorber.
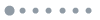
Figure 2.Schematic of the experimental setup.
By ABCD matrix calculation, the laser beam waist in the gain medium is calculated to be , which matches the pump laser mode well. The laser mode size focused on the BP SA is estimated as . A fast photodetector (DET10 A/M, Thorlabs, Inc., U.S.) with a specified rise time of 1 ns and a digital oscilloscope (TDS5000B, Tektronix, Inc., U.S.) with 1 GHz bandwidth are employed to detect and record the laser pulse in the experiment.
First, we replace the BP solution SA by using an HR mirror and obtain a continuous wave (CW) laser operation. As shown in Fig. 3(a), black squares and black line represent the average output power of the CW laser and fitting line, respectively. The threshold of the pump power for CW operation is 3 W. The maximum average output power is 6 W under a pump power of 16 W, corresponding to a slope efficiency of 46.2%. Then, replacing the HR mirror with the BP solution SA in the same laser resonator, the passively QS operation is realized by finely tuning the position of the SA in the laser cavity and adjusting the pump power. Such QS is observed when the pump power rises to 12 W, and it can be maintained as the pump power rises up to 16 W. For QS operation, the evolution of the average output power with the pump power is demonstrated by the blue pentagram notations and fitting line of Fig. 3(a). The slope efficiency is 12.6% and the maximum average output power is 1.23 W, which is the highest output power among pulse lasers based on BP and other graphene-like 2D nanomaterials so far. Such high output power is attributed to the high damage resistance of the BP solution SA. As mentioned in Table 1, most of the reported graphene-like 2D nanomaterial absorbers are exposed to the air, and these materials have a low laser damage threshold. Thus, all the reported average output power is below 1 W. Different from these solid state SAs, our BP solution SA can effectively dissipate heat into the solvent, which increases the laser damage resistance of the 2D materials and helps the absorber to withstand high power laser irradiation. It can be found that the threshold of the QS is much higher and the slope efficiency of the QS laser is far less than that of the CW laser, which mainly attributes to the insertion loss of the BP solution SA, including the refraction loss generated on the surfaces of the quartz cell and the nonsaturable loss of the BP SA.
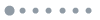
Figure 3.(a) Output power versus the pump power. (b) The pulse trains obtained at different pump powers. (c) Evolutions of the pulse repetition rate and of the pulse width with the pump power. (d) Single pulse profile of the QS laser. (e) Optical spectrum.
The laser operates at the CW state when the pump power is below 12 W and at the QS state when the pump power is above 12 W. The QS become unstable when the pump power exceeds 16 W. The oscilloscope traces are measured at the pump power of 11, 12, 13, 14, 15, and 16 W, respectively, which are shown in Fig. 3(b). The evolutions of the pulse repetition rate and pulse width with the pump power are exhibited in Fig. 3(c). As the pump power increases from 12 to 16 W, the pulse repetition rate increases continuously from 533.2 to 722 kHz while the pulse width decreases from 230 to 119 ns. The latter is narrower than all those reported recently in BP-based QS SSLs[29–32]. The profile of the pulse is shown in Fig. 3(d). As shown in Fig. 3(e), the optical spectrum of the QS laser is centered at 1064.4 nm and the full width at half-maximum is measured to be 0.132 nm. During the experiment, the QS pulse trains are stable and the BP SA are effective all the time, while no damage on the SA solution has been observed. The results demonstrate that the BP solution SA possesses a high damage resistance and can be used for a high power passively -switching operation.
In conclusion, we demonstrate a diode end-pumped passively QS laser at 1064 nm with a high damage threshold BP solution SA. The shortest pulse duration is 119 ns, corresponding to a repetition rate of 722 kHz. The maximum average output power is 1.23 W corresponding to a slope efficiency of 12.6%. The results illustrate that the BP solution SAs have the potential to apply to high-power pulse lasers.
References
[1] T. T. Kajava, A. L. Gaeta. Opt. Lett., 21, 1244(1996).
[2] U. Keller, K. J. Weingarten, F. X. Kärtner, D. Kopf, B. Braun, I. D. Jung, J. A. D. Au. IEEE J. Sel. Top. Quantum Electron., 2, 435(1996).
[3] Z. Sun, T. Hasan, F. Torrisi, D. Popa, G. Privitera, F. Wang, F. Bonaccorso, D. M. Basko, A. C. Ferrari. ACS Nano., 4, 803(2010).
[4] Y. G. Wang, H. R. Chen, X. M. Wen, W. F. Hsieh, J. Tang. Nanotechnology, 22, 455203(2011).
[5] X. L. Li, J. L. Xu, Y. Z. Wu, J. L. He, X. P. Hao. Opt. Express, 19, 9950(2011).
[6] Y. G. Wang, H. R. Chen, W. F. Hsieh, Y. H. Tsang. Opt. Commun., 289, 119(2013).
[7] Q. Wen, X. J. Zhang, Y. G. Wang, Y. S. Wang, H. B. Niu. IEEE Photon. J., 6, 1(2014).
[8] H. Zhang, C. X. Liu, X. L. Qi, X. Dai, Z. Fang, S. C. Zhang. Nat. Phys., 5, 438(2009).
[9] F. Q. Jia, H. Chen, P. Liu, Y. Z. Huang, Z. Q. Luo. IEEE J. Sel. Top. Quantum Electron., 21, 1601806(2015).
[10] H. S. S. Ramakrishna Matte, A. Gomathi, A. K. Manna, D. J. Late, R. Datta, S. K. Pati, C. N. R. Rao. Angew. Chem., 122, 4153(2010).
[11] K. P. Wang, Y. Y. Feng, C. X. Chang, J. X. Zhan, C. W. Wang, Q. Z. Zhao, J. N. Coleman, L. Zhang, W. J. Blauab, J. Wang. Nanotechnology, 6, 10530(2014).
[12] Y. J. Sun, C. K. Lee, J. L. Xu, Z. J. Zhu, Y. Q. Wang, S. F. Gao, H. P. Xia, Z. Y. You, C. Y. Tu. Photon. Res., 3, A97(2015).
[13] J. N. Coleman, M. Lotya, A. O’Neill, S. D. Bergin, P. J. King, U. Khan, I. V. Shvets. Science, 331, 568(2011).
[14] L. Li, S. Z. Jiang, Y. G. Wang, X. Wang, L. N. Duan, D. Mao, Z. Li, B. Y. Man, J. H. Si. Opt. Express, 23, 28934(2015).
[15] B. Xu, Y. J. Cheng, Y. Wang, Y. Z. Huang, J. Peng, Z. Q. Luo, H. Y. Xu, Z. P. Cai, J. Weng, R. Moncorgé. Opt. Express, 22, 28934(2014).
[16] P. G. Ge, J. Liu, S. Z. Jiang, Y. Y. Xu, B. Y. Man. Photon. Res., 3, 256(2015).
[17] X. Zou, Y. Leng, Y. Li, Y. Feng, P. Zhang, Y. Hang, J. Wang. Chin. Opt. Lett., 13, 081405(2015).
[18] F. Lou, R. W. Zhao, J. L. He, Z. T. Jia, X. C. Su, Z. W. Wang, J. Hou, B. T. Zhang. Photon. Res., 3, A25(2015).
[19] Y. X. Zhang, S. X. Wang, H. H. Yu, H. J. Zhang, Y. X. Chen, L. M. Mei, A. D. Lieto, M. Tonelli, J. Y. Wang. Sci. Rep., 5, 11342(2015).
[20] J. Hou, G. Zhao, Y. Z. Wu, J. L. He, X. P. Hao. Opt. Express, 23, 27292(2015).
[21] C. Feng, X. Y. Zhang, J. Wang, Z. J. Liu, Z. H. Cong, H. Rao, Q. P. Wang, J. X. Fang. Opt. Mater. Express, 6, 1358(2016).
[22] X. Wang, Y. G. Wang, L. N. Duan, L. Li, H. Sun. Opt. Commun., 367, 234(2016).
[23] R. C. T. Howe, G. H. Hu, Z. Y. Yang, T. Hasan. Low-Dimens. Mater. Devices, 95530, R1(2015).
[24] J. Sotor, G. Sobon, M. Kowalczyk, W. Macherzynski, P. Paletko, K. M. Abramski. Opt. Lett., 40, 3885(2015).
[25] S. B. Lu, L. L. Miao, Z. N. Guo, X. Qi, C. J. Zhao, H. Zhang, S. C. Wen, D. Y. Tang, D. Y. Fan. Opt. Express, 23, 11183(2015).
[26] R. Zhang, Y. X. Zhang, H. H. Yu, H. J. Zhang, R. N. Yang, B. C. Yang, Z. Y. Liu, J. Y. Wang. Adv. Opt. Mater., 3, 1787(2015).
[27] L. C. Kong, Z. P. Qin, G. Q. Xie, Z. N. Guo, H. Zhang, P. Yuan, L. J. Qian. Laser Phys. Lett., 13, 045801(2016).
[28] P. X. Li, G. J. Zhang, H. Zhang, C. J. Zhao, J. J. Chi, Z. Q. Zhao, C. Yang, H. W. Hu, Y. F. Yao. IEEE Photon. Technol. Lett., 26, 1912(2014).
[29] X. C. Su, Y. R. Wang, B. T. Zhang, R. W. Zhao, K. J. Yang, J. L. He, Q. Q. Hu, Z. T. Jia, X. T. Tao. Opt. Lett., 41, 1945(2016).
[30] D. Z. Lu, Z. B. Pan, R. Zhang, T. X. Xu, R. L. Yang, B. C. Yang, Z. Y. Liu, H. H. Yu, H. J. Zhang, J. Y. Wang. Opt. Eng., 55, 081312(2016).
[31] Z. W. Wang, R. W. Zhao, J. L. He, B. T. Zhang, J. Ning, Y. R. Wang, X. C. Su, J. Hou, F. Lou, K. J. Yang, Y. S. Fan, J. T. Bian, J. S. Nie. Opt. Express, 24, 1598(2016).
[32] B. T. Zhang, F. Lou, R. W. Zhao, J. L. He, J. Li, X. C. Su, J. Ning, K. J. Yang. Opt. Lett., 40, 3691(2015).
[33] J. H. Liu, J. R. Tian, M. T. Hu, Z. Y. Dou, Y. R. Song. Chin. J. Lasers, 42, 0802004(2015).
[34] J. L. Xu, C. Y. Tu, Y. J. Chiu, C. K. Lee. IEEE Photonics Conference, 406(2014).
[35] H. H. Yu, H. Zhang, Y. C. Wang, C. J. Zhao, B. l. Wang, S. C. Wen, H. J. Zhang, J. Y. Wang. Laser Photon. Rev., 7, L77(2013).