
- Chinese Optics Letters
- Vol. 20, Issue 9, 090501 (2022)
Abstract
1. Introduction
Optical computing has again garnered significant attention in the past few years, due to its potentials in addressing the barriers and challenges in computing power and energy efficiency encountered by conventional electronic processors in the post Moore era[
Currently, the grating couplers for planar substrates are mainly realized by blazed or slanted gratings. The utilization of blazed gratings is the most straightforward choice to realize the deflection of light. However, for conventional blazed gratings, the deflection angle is generally not large enough, and the coupling efficiency is also not very high (typically 84%)[
Sign up for Chinese Optics Letters TOC. Get the latest issue of Chinese Optics Letters delivered right to you!Sign up now
In this study, a high-efficiency broadband grating coupler for vertical coupling based on an asymmetric double-groove structure is designed using the rigorous coupled wave algorithm (RCWA) and simulated annealing (SA) algorithm. The asymmetric structure allows perfect vertical fiber coupling, resulting in less difficulties in alignment and packaging, less angle dependence, and denser integration. It is indicated that the average coupling efficiency of the
2. Design Consideration and Optimization Model
2.1. Design consideration
Figure 1 illustrates the structure diagram of the asymmetric double-groove grating coupler. A TE-polarized plane wave is normally incident from top to bottom. The cover medium is air, and the refractive index of
Figure 1.Schematic diagram of the grating coupler based on the double-groove structure. The green arrow represents the polarization direction, port #1 represents the reflective 1st order, and ports #2, #3, and #4 represent the transmitted +1st, 0th, and −1st orders, respectively.
According to the analysis results of the mode coupling theory[
2.2. Optimization model
Based on the above discussions, the RCWA method[
3. Results and Discussions
The final optimization results of the six structure parameters in the C and C+L bands are presented in Table 1. It is evident that the grating period is
Band | ||||||||
---|---|---|---|---|---|---|---|---|
C (1535–1565 nm) | 0.331 | 0.29 | 0.147 | 0.873 | 1.109 | 1.467 | 97.3 | 1.5 |
C+L (1535–1625 nm) | 0.341 | 0.27 | 0.148 | 0.893 | 1.137 | 1.476 | 92.8 | 1.6 |
Table 1. Structure Parameters and Diffraction Efficiencies of Two Double-Groove Grating Couplers Optimized for TE Polarization Working in the C and C+L Bands, Respectively
Furthermore, we simulated the electric field distribution based on the finite element method (FEM), when a collimated Gaussian beam from a single-mode fiber with a collimation lens is normally incident on the grating coupler with TE polarization. Here, the waist radius of the collimated Gaussian beam is 75 µm with a monochromatic wavelength of 1550 nm, and the incident electric field power is 1 V/m. The upper and lower boundary conditions of the substrate are defined as impedance boundary conditions, and other boundary conditions are defined as scattering boundary conditions. A self-adaptive triangular mesh is selected, and the maximum mesh size is 200 nm. The simulation results illustrated in Fig. 2 clearly indicate that light energy is dominantly coupled into the
Figure 2.Simulation results of the electric field inside the substrate with the double-groove grating coupler when the Gaussian wave from a single-mode fiber is normally incident with TE polarization. The inset (in the red box) is the enlarged electric field distribution within the grating region.
In addition, the diffraction efficiency versus the wavelength is investigated further. As illustrated in Fig. 3(a), the diffraction efficiency of the
Figure 3.Relationship between the coupling efficiency calculated by the RCWA, SA algorithms as well as FEM, and the working wavelength λ (a) in the C band and (b) in the C+L band, and (c) electric field distribution diagram at the central wavelength of λ = 1550 nm.
4. Tolerance Analysis
In the manufacturing process of the grating coupler, the actual shape of the grating structure always deviates from optimized parameters because of various fabrication errors, resulting in the deterioration in performances, such as coupling efficiency, and light noises at other undesired orders of the grating coupler. Therefore, it is necessary to assess the manufacturing tolerances of the grating design. In this section, we have selected several important structure parameters of the grating coupler for tolerance investigation.
When other parameters are fixed and equal to the optimized parameters, the influence of each parameter, including the grating period
In the C band, it is observed from Fig. S1(a) in Supplementary Material that the grating period
In the C+L band, as shown in Fig. S2 in Supplementary Material, it is evident that a tolerance of 35 nm, 30 nm, 13 nm, 12 nm, 14 nm, and 265 nm is obtained for
Figure 4.Simulation results of 1000 samples of the grating couplers based on the Monte Carlo method. The average efficiencies of the couplers in the (a) C and (b) C+L bands for these 1000 samples; statistical distributions of the efficiency of these 1000 samples in the (c) C and (d) C+L bands.
These analyses show that the proposed double-groove grating coupler has good tolerance, and these couplers should be realizable by standard e-beam lithography and plasma-based dry etching technologies. The coupling efficiency is far larger than that of the couplers in nano-waveguides, and the double-groove grating couplers could be used in the wideband. Moreover, note that the tolerance of the refractive index of two ridge layers, especially the second layer, is very large, which suggests that the derivation in the refractive index has little influence on the coupling efficiency. Therefore, it is expected to construct PIOS-based integrated optoelectronic intelligent systems based on different material systems.
5. Conclusion
Based on the RCWA and SA algorithm, a type of double-groove grating for high-efficiency wideband vertical coupling in PIOSs was designed. As examples, two double-groove gratings were designed for the C and C+L bands, respectively. The average coupling efficiency of the
References
[1] M. A. Taubenblatt. Optical interconnects for high-performance computing. J. Light. Technol., 30, 448(2012).
[2] G. Wetzstein, A. Ozcan, S. Gigan, S. Fan, D. Englund, M. Soljačić, C. Denz, D. Miller, D. Psaltis. Inference in artificial intelligence with deep optics and photonics. Nature, 588, 39(2020).
[3] W. Bogaerts, D. Pérez, J. Capmany, D. Miller, J. Poon, D. Englund, F. Morichetti, A. Melloni. Programmable photonic circuits. Nature, 586, 207(2020).
[4] C. Li, X. Zhang, J. Li, T. Fang, X. Dong. The challenges of modern computing and new opportunities for optics. PhotoniX, 2, 20(2021).
[5] Y. Shen, N. Harris, S. Skirlo, M. Prabhu, T. Baehr-Jones, M. Hochberg, X. Sun, S. Zhao, H. Larochelle, D. Englund, M. Soljačić. Deep learning with coherent nanophotonic circuits. Nat. Photonics, 11, 441(2017).
[6] J. Feldmann, N. Youngblood, M. Karpov, H. Gehring, X. Li, M. Stappers, M. Le Gallo, X. Fu, A. Lukashchuk, A. S. Raja, J. Liu, C. D. Wright, A. Sebastian, T. J. Kippenberg, W. H. P. Pernice, H. Bhaskaran. Parallel convolutional processing using an integrated photonic tensor core. Nature, 589, 52(2021).
[7] B. Shastri, A. Tait, T. Lima, W. Pernice, H. Bhaskaran, C. Wright, P. Prucnal. Photonics for artificial intelligence and neuromorphic computing. Nat. Photonics, 15, 102(2021).
[8] X. Lin, Y. Rivenson, N. T. Yardimci, M. Veli, M. Jarrahi, A. Ozcan. All-optical machine learning using diffractive deep neural networks. Science, 361, 1004(2018).
[9] T. Zhou, L. Fang, T. Yan, J. Wu, Y. Li, J. Fan, H. Wu, X. Lin, Q. Dai. In situ optical backpropagation training of diffractive optical neural networks. Photonics Res., 8, 940(2020).
[10] T. Zhou, X. Lin, J. Wu, Y. Chen, H. Xie, Y. Li, J. Fan, H. Wu, L. Fang, Q. Dai. Large-scale neuromorphic optoelectronic computing with a reconfigurable diffractive processing unit. Nat. Photonics, 15, 367(2021).
[11] A. Ryou, J. Whitehead, M. Zhelyeznyakov, P. Anderson, C. Keskin, M. Bajcsy, A. Majumdar. Free-space optical neural network based on thermal atomic nonlinearity. Photonics Res., 9, B128(2021).
[12] D. Pierangeli, G. Marcucci, C. Conti. Photonic extreme learning machine by free-space optical propagation. Photonics Res., 9, 1446(2021).
[13] G. Matthias, J. Jürgen, S. Stefan. Planar-integrated optical vector-matrix multiplier. Appl. Opt., 39, 5367(2000).
[14] M. Gladys, G. Matthias, J. Jürgen, L. Jesús. Achromatic optical Fourier transformer with planar-integrated free-space optics. Appl. Opt., 44, 229(2005).
[15] R. Heming, L. Wittig, P. Dannberg, J. Jahns, E. Kley, M. Gruber. Efficient planar-integrated free-space optical interconnects fabricated by a combination of binary and analog lithography. J. Light. Technol., 26, 14(2008).
[16] H. Ma, H. Yang, B. Tang, M. Wei, J. Li, J. Wu, P. Zhang, C. Sun, L. Li, H. Lin. Passive devices at 2 µm wavelength on 200 mm CMOS-compatible silicon photonics platform [Invited]. Chin. Opt. Lett., 19, 071301(2021).
[17] R. Ge, H. Li, Y. Han, L. Chen, J. Xu, M. Wu, Y. Li, Y. Luo, X. Cai. Polarization diversity two-dimensional grating coupler on x-cut lithium niobate on insulator. Chin. Opt. Lett., 19, 060006(2021).
[18] N. Hoppe, W. Zaoui, L. Rathgeber, Y. Wang, R. Klenk, W. Vogel, M. Kaschel, S. Portalupi, J. Burghartz, M. Berroth. Ultra-efficient silicon-on-insulator grating couplers with backside metal mirrors. IEEE J. Quantum Electron., 26, 0001801(2020).
[19] J. Xiong, E. Hsiang, Z. He, T. Zhan, S. Wu. Augmented reality and virtual reality displays: emerging technologies and future perspectives. Light Sci. Appl., 10, 216(2021).
[20] M. Tian, M. Qu, L. Wu, X. Cheng. Progress on asymmetrical grating couplers for vertical coupling. Laser Optoelectron. Prog., 58, 0500004(2021).
[21] J. N. Mait, D. W. Prather, M. S. Mirotznik. Design of binary subwavelength diffractive lenses by use of zeroth-order effective-medium theory. J. Opt. Soc. Am. A, 16, 1157(1999).
[22] J. Xu, S. Yang, L. Wu, L. Xu, Y. Li, R. Liao, M. Qu, X. Quan, X. Cheng. Design and fabrication of a high-performance binary blazed grating coupler for perfectly perpendicular coupling. Opt. Express, 29, 42999(2021).
[24] Y. Ding, R. Magnusson. Resonant leaky-mode spectral-band engineering and device applications. Opt. Express, 12, 5661(2004).
[25] L. Sun, Z. Lv, W. Wu, W. Liu, J. Yuan. Double-grating polarizer for terahertz radiation with high extinction ratio. Appl. Opt., 49, 2066(2010).
[26] J. Wu, C. Zhou, H. Cao, A. Hu, J. Yu, W. Sun, W. Jia. Beam splitting of a double-groove fused-silica grating under normal incidence. J. Opt., 13, 115703(2011).
[27] K. Ito, H. Lizuka. Highly efficient -1st-order reflection in Littrow mounted dielectric double-groove grating. AIP Adv., 3, 062119(2013).
[28] J. Wu. Enhanced light trapping with double-groove grating in thin-film amorphous silicon solar cells. Opt. Laser Tech., 79, 95(2016).
[29] Y. Liu, J. Feng, Z. Li, J. Luo, T. Kou, S. Yuan, M. Li, Y. Liu, Y. Peng, S. Wang. Double-groove terahertz chirped grating waveguide tube for gas pressure detection. Laser Phys. Lett., 16, 056202(2019).
[30] P. Laakkonen, S. Siitonen, M. Kuittinen. Double-groove, two-depth grating coupler for light guides. J. Opt. Soc. Am. A., 23, 3156(2006).
[31] M. Oliva, D. Michaelis, T. Benkenstein, J. Dunkel, J. Harzendorf, A. Matthes, U. D. Zeitner. Highly efficient three-level blazed grating in the resonance domain. Opt. Lett., 35, 2774(2010).
[32] H. Iizuka, N. Engheta, H. Fujikawa, K. Sato, Y. Takeda. Role of propagating modes in a double-groove grating with a +1st-order diffraction angle larger than the substrate–air critical angle. Opt. Lett., 35, 3973(2010).
[33] T. Matsui, A. Miura, N. Ikeda, H. Fujikawa, Y. Sugimoto, N. Engheta, H. Iizuka. Experimental investigation of double-groove grating satisfying total internal reflection condition. Opt. Express., 22, 25362(2014).
[34] D. T. Pierce, W. E. Spicer. Electronic structure of amorphous Si from photoemission and optical studies. Phys. Rev. B, 5, 3017(1972).
[35] T. Levola. Diffractive optics for virtual reality displays. J. Soc. Inf. Disp., 14, 467(2006).
[36] H. Mukawa. A full-color eyewear display using planar waveguides with reflection volume holograms. J. Soc. Inf. Disp., 17, 185(2009).
[37] Z. Liu, C. Pan, Y. Pang, Z. Huang. A full-color near-eye augmented reality display using a tilted waveguide and diffraction gratings. Opt. Commun., 431, 45(2019).
[38] M. G. Moharam, T. K. Gaylord. Rigorous coupled-wave analysis of planar-grating diffraction. J. Opt. Soc. Am., 71, 811(1981).
[39] M. G. Moharam, Eric B. Grann, Drew A. Pommet, T. K. Gaylord. Formulation for stable and efficient implementation of the rigorous coupled-wave analysis of binary gratings. J. Opt. Soc. Am. A, 12, 1068(1995).
[40] X. Xiang, M. Li, C. Wei, C. Zhou. Precision fringe period metrology using an LSQ sine fit algorithm. Appl. Opt., 57, 4777(2018).
[41] W. C. Chuang, C. T. Ho, W. C. Wang. Fabrication of a high-resolution periodical structure using a replication process. Opt. Express, 13, 6685(2005).
[42] K. J. Byeon, H. Lee. Recent progress in direct patterning technologies based on nano-imprint lithography. EPJ Appl. Phys., 59, 10001(2012).
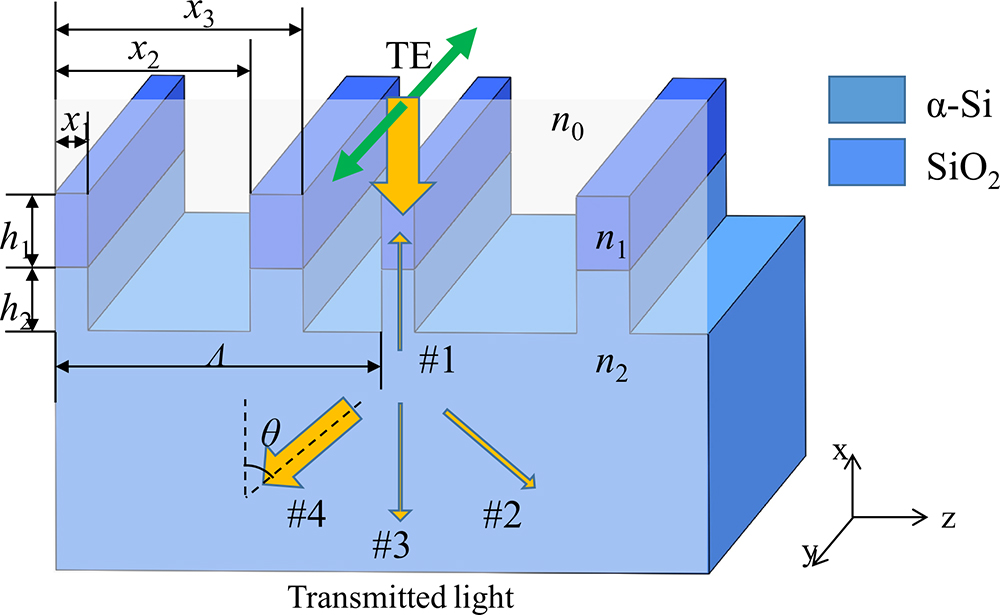
Set citation alerts for the article
Please enter your email address