
- Chinese Optics Letters
- Vol. 21, Issue 1, 011404 (2023)
Abstract
1. Introduction
Enabling the construction of complex optoelectronic systems in a reliable and cost-effective way, the potential of photonic integrated circuits (PICs) is now explored in many applications, such as optical communication[1,2], computing[3], sensing[4], and detecting[5]. There has been substantial progress achieved in recent years, however, transmission loss resulting from absorption, reflection, diffraction, scattering, or coupling efficiency remains a key challenge to improve the functionalities of integrated devices[6–8]. Limited by the fabrication technologies as well as material platforms, the attenuation of light energy at the interface is inevitable and not negligible, which should be compensated by optical amplification to ensure adequate power efficiency, signal noise ratio, or quality factor.
Inspired by the great success of erbium-doped fiber amplifiers (EDFAs) in optical telecommunication, erbium-doped waveguide amplifiers (EDWAs) have attracted much attention in decades[9]. Compared to semiconductor optical amplifiers (SOAs) based on electron–hole pairs recombination in III–V semiconductors, EDWAs can offer longer excited-state lifetime, simpler structure, lower noise, broader bandwidth, and thermally stabler optical gain, allowing for high-speed amplification[9,10]. Despite the fact that Er ions interactions and clustering effects constrain the dopant concentration, highly
Slot waveguides are of high interest in integrated optics owing to their ability to confine light strongly in a very thin low-refractive-index region. For this reason, they have shown great potential to produce high-performance integrated waveguide lasers and amplifiers[16–19]. But, a vertical slot fabrication involves etching in a very narrow region, which can cause severe roughness in the waveguide sidewall in contrast to the precise and easy height control of the deposition layer in current micro/nanofabrication technologies; as a result, a horizontal slot is more favorable in the EDWA design for its lower propagation loss[20,21]. In this paper, a 40 nm thick horizontal slot of
Sign up for Chinese Optics Letters TOC. Get the latest issue of Chinese Optics Letters delivered right to you!Sign up now
Figure 1.Structure of slot waveguide. (a) Two-dimensional schematic of horizontal slot waveguide; (b) three-dimensional schematic of horizontal slot waveguide.
2. Design of the Slot Waveguide
Since active material exists in the slot region only, a waveguide in which pump and signal light is more concentrated in the low-refractive-index area is desired for higher energy availability. Also, a higher electric field intensity in the active material is needed for a stronger light–matter interaction. The trade-off of these two requirements implies that the structure of the slot waveguide used for amplification should be carefully designed, according to the mode distribution. In this work, the COMSOL software based on the finite element method (FEM) was used to simulate the modal distribution and optimize the waveguide structure. A two-dimensional model was built in the Wave Optics Module, coupled with the Electromagnetic Waves Frequency Domain (ewfd) interface, and studied by Mode Analysis solver. The schematics of the slot waveguide with horizontal sidewalls are displayed in Fig. 1. An image of the cross section of the sandwiched waveguide is shown in Fig. 1(a), and that in the 3D view is shown in Fig. 1(b). An Er-doped
Considering the overlap between the signal and pump beam, the modal profile should be simulated at both
Figure 2.Distribution of normalized |E (x, y)| in slot waveguide with the Si guiding layer height hg = 200 nm, width w = 300 nm, and slot height hs = 40 nm. (a) TM mode profile at 1530 nm; (b) TM mode profile at 1480 nm; (c) TE mode profile at 1530 nm; (d) TE mode profile at 1480 nm. The white arrows represent the directions and amplitudes of the electric field in the xy plane.
Material | Index at 1530 nm | Index at 1480 nm |
---|---|---|
Si | 3.478 | 3.482 |
1.444 | 1.451 | |
2.06 | 2.07 |
Table 1. Refractive Indices Used in Simulation[22,23]
To coordinate the two requirements in a more quantitative way, we define two figures of merit as follows: the light field confinement factor (
As a prominent factor of slot waveguide functionality, the value of slot height
Figure 3.Simulation results for varying hs from 10 to 120 nm. (a) Effective refractive indices neff of the TM/TE mode at 1530 nm and 1480 nm; (b) confinement factors Γs of the TM/TE mode at 1530 nm and 1480 nm; (c) normalized power density Inorm of the TM/TE mode at 1530 nm and 1480 nm.
The silicon layer height
Figure 4.Optimization of width and height of the Si layer with a 40 nm thick slot. (a) Field confinement factors Γs as a function of single silicon layer height hg and waveguide width w. (b) Normalized power density Inorm as a function of single silicon layer height hg and waveguide width w. The white dashed lines in (a) and (b) are the boundaries of the neff (TM) > neff (TE) condition; only the domains below these lines are valid. The white dots in (a) and (b) denote Γs = 29.17% and Inorm = 24.31 µm−2, respectively, at the positions of hg = 200 nm and w = 300 nm.
3. Gain Characteristics of Slot Waveguide Amplifier
Supported by the optimization of the waveguide, the Er-doped low-refractive-index material is filled into the slot waveguide, and the potential gain of the slot waveguide amplifier is investigated. The gain characteristic analysis is based on the
Figure 5.Energy-level transitions for the Er3+-doped four-level system (pumped by 1480 nm).
The meanings and the values of the relevant parameters are shown in Table 2. Because it is difficult to test accurately all the parameters of the active material used in the simulation, the values in Table 2 are mainly accumulated from recent researches[11,29]. The
Figure 6.Simulated gain characteristics of the designed slot waveguide amplifier. (a) Gain versus propagation distance for different pump powers. (b) Gain versus pump power for different amplifier lengths. For both situations, the signal power is assumed to be 0.01 mW.
Parameter | Full name | Value |
---|---|---|
NEr | Erbium ion density | |
Absorption cross section at 1480 nm | ||
Emission cross section at 1480 nm | ||
Excited-state absorption cross section | ||
Absorption cross section at 1530 nm | ||
Emission cross section at 1530 nm | ||
A21 | Spontaneous emission rate of N2 | |
A32 | Spontaneous emission rate of N3 | |
A43 | Spontaneous emission rate of N4 | |
Cup | Up-conversion coefficient | |
C14 | Cross-relaxation coefficient | |
Lp | Propagation loss of the pump laser | 1 dB/cm |
Ls | Propagation loss of the signal laser | 1 dB/cm |
Table 2. Parameter Values of TeO2:Er3+ Rate Equation Model[11,23]
To study the dynamics of the amplification process where the pump and signal light are input to a slot waveguide in the same direction, the change of signal power propagating in the amplifier along with pump light is shown in Fig. 6(a). As can be seen, the signal gain functions versus propagation length under 0.1, 1, and 10 mW pumping are similar: the signal power increases at first and then decreases continually, and the length shows an optimum value. The mechanism is that the gain and loss are two competitive processes in the signal light transmission, the former stemming from the stimulated emission and the latter from absorption and propagation loss in the waveguide. Therefore, population inversion is critical to steer the net effect, which depends on whether the pump power is high enough to resist various de-population processes of the
4. Conclusions
Waveguide amplifiers have always been significant components for integrated photonics. Unfortunately, the low concentration of rare earth ions doped in the host and the inadequate optimization of the waveguide structure have been the common bottleneck. In our work, the performance of the horizontal slot waveguide amplifier based on
References
[1] K. Liu, H. Fan, Y. Huang, X. Duan, Q. Wang, X. Ren, Q. Wei, S. Cai. A pair of integrated optoelectronic transceiving chips for optical interconnects. Chin. Opt. Lett., 16, 091301(2018).
[2] E. Agrell, M. Karlsson, A. R. Chraplyvy, D. J. Richardson, P. M. Krummrich, P. Winzer, K. Roberts, J. K. Fischer, S. J. Savory, B. J. Eggleton, M. Secondini, F. R. Kschischang, A. Lord, J. Prat, I. Tomkos, J. E. Bowers, S. Srinivasan, M. Brandt-Pearce, N. Gisin. Roadmap of optical communications. J. Opt., 18, 063002(2016).
[3] W. Bogaerts, D. Pérez, J. Capmany, D. A. B. Miller, J. Poon, D. Englund, F. Morichetti, A. Melloni. Programmable photonic circuits. Nature, 586, 207(2020).
[4] A. Hänsel, M. J. R. Heck. Opportunities for photonic integrated circuits in optical gas sensors. J. Phys. Photonics, 2, 12002(2020).
[5] J. Fridlander, F. Sang, V. Rosborough, F. Gambini, S. T. Šuran-Brunelli, J. R. Chen, K. Numata, M. Stephen, L. A. Coldren, J. Klamkin. Dual laser indium phosphide photonic integrated circuit for integrated path differential absorption lidar. IEEE J. Sel. Top. Quantum Electron., 28, 6100208(2022).
[6] A. Rahim, A. Hermans, B. Wohlfeil, D. Petousi, B. Kuyken, D. V. Thourhout, R. Baets. Taking silicon photonics modulators to a higher performance level: state-of-the-art and a review of new technologies. Adv. Photonics, 3, 024003(2021).
[7] L. Lu, S. Zhao, L. Zhou, D. Li, Z. Li, M. Wang, X. Li, J. Chen. Non-blocking silicon optical switch based on electro-optic Mach-Zehnder interferometers. Opt. Express, 24, 9295(2016).
[8] L. He, Ş. K. Özdemir, L. Yang. Whispering gallery microcavity lasers. Laser Photon. Rev., 7, 60(2012).
[9] J. D. B. Bradley, M. Pollnau. Erbium-doped integrated waveguide amplifiers and lasers. Laser Photon. Rev., 5, 368(2010).
[10] D. R. Zimmerman, L. H. Spiekman. Amplifiers for the masses: EDFA, EDWA, and SOA amplets for metro and access applications. J. Light. Technol., 22, 63(2004).
[11] H. C. Frankis, H. M. Mbonde, D. B. Bonneville, C. Zhang, R. Mateman, A. Leinse, J. D. B. Bradley. Erbium-doped TeO2-coated Si3N4 waveguide amplifiers with 5 dB net gain. Photon. Res., 8, 127(2020).
[12] J. S. Wang, E. M. Vogel, E. Snitzer. Tellurite glass: a new candidate for fiber devices. Opt. Mater., 3, 187(1994).
[13] A. Mori, Y. Ohishi, S. Sudo. Erbium-doped tellurite glass fibre laser and amplifier. Electron. Lett., 33, 863(1997).
[14] Y. Ohishi, A. Mori, M. Yamada, H. Ono, Y. Nishida, K. Oikawa. Gain characteristics of tellurite-based erbium-doped fiber amplifiers for 1.5-µm broadband amplification. Opt. Lett., 23, 274(1998).
[15] S. Shen, A. Jha, X. Liu, M. Naftaly, K. Bindra, H. J. Bookey, A. K. Kar. Tellurite glasses for broadband amplifiers and integrated optics. J. Am. Ceram. Soc., 85, 1391(2002).
[16] V. R. Almeida, Q. Xu, C. A. Barrios, M. Lipson. Guiding and confining light in void nanostructure. Opt. Lett., 29, 1209(2004).
[17] J. T. Robinson, C. Manolatou, L. Chen, M. Lipson. Ultrasmall mode volumes in dielectric optical microcavities. Phys. Rev. Lett., 95, 143901(2005).
[18] C. A. Barrios, M. Lipson. Electrically driven silicon resonant light emitting device based on slot-waveguide. Opt. Lett., 13, 10092(2005).
[19] M. Galli, D. Gerace, A. Politi, M. Liscidini, M. Patrini, L. C. Andreani, A. Canino, M. Miritello, R. L. Savio, A. Irrera, F. Priolo. Direct evidence of light confinement and emission enhancement in active silicon-on-insulator slot waveguides. Appl. Phys. Lett., 89, 241114(2006).
[20] R. Sun, P. Dong, N. Feng, C. Hong, J. Michel, M. Lipson, L. Kimerling. λ = 1550 nm horizontal single and multiple slot waveguides: optical transmission at λ = 1550 nm. Opt. Express, 15, 17967(2007).
[21] A. Tengattini, D. Gandolfi, N. Prtljaga, A. Anopchenko, J. M. Ramirez, F. F. Lupi, Y. Berencen, D. Navarro-Urrios, P. Rivallin, K. Surana, B. Garrido, J.-M. Fedeli, L. Pavesi. Toward a 1.54 µm electrically driven erbium-doped silicon slot waveguide and optical amplifier. J. Light. Technol., 31, 391(2013).
[22] V. A. G. Rivera, D. Manzani. Technological Advances in Tellurite Glasses: Properties, Processing, and Applications(2017).
[23] M. Zhang, G. Hu, S. Zhang, D. Gao, Y. Sun, F. Wang. Gain characteristics of the hybrid slot waveguide amplifiers integrated with NPs-PMMA covalently linked nanocomposites. RSC Adv., 10, 11148(2020).
[24] R. A. H. El-Mallawany. Tellurite Glasses Handbook: Physical Properties and Data(2016).
[25] T. D. Visser, H. Blok, B. Demeulenaere, D. Lenstra. Confinement factors and gain in optical amplifiers. IEEE J. Quantum Electron., 33, 1763(1997).
[26] N. Feng, J. Michel, L. C. Kimerling. Optical field concentration in low-index waveguides. IEEE J. Quantum Electron., 42, 883(2006).
[27] P. Mullner, R. Hainberger. Structural optimization of silicon-on-insulator slot waveguides. IEEE Photon. Technol. Lett., 18, 2557(2006).
[28] P. Sanchis, J. Blasco, A. Martinez, J. Marti. Design of silicon-based slot waveguide configurations for optimum nonlinear performance. J. Light. Technol., 25, 1298(2007).
[29] Y. Hu, S. Jiang, G. Sorbello, T. Luo, Y. Ding, B. Hwang, J. Kim, H. Seo, N. Peyghambarian. Cooperative upconversion in a new high-Er-doped tellurite glass. Proc. SPIE, 4282, 57(2001).
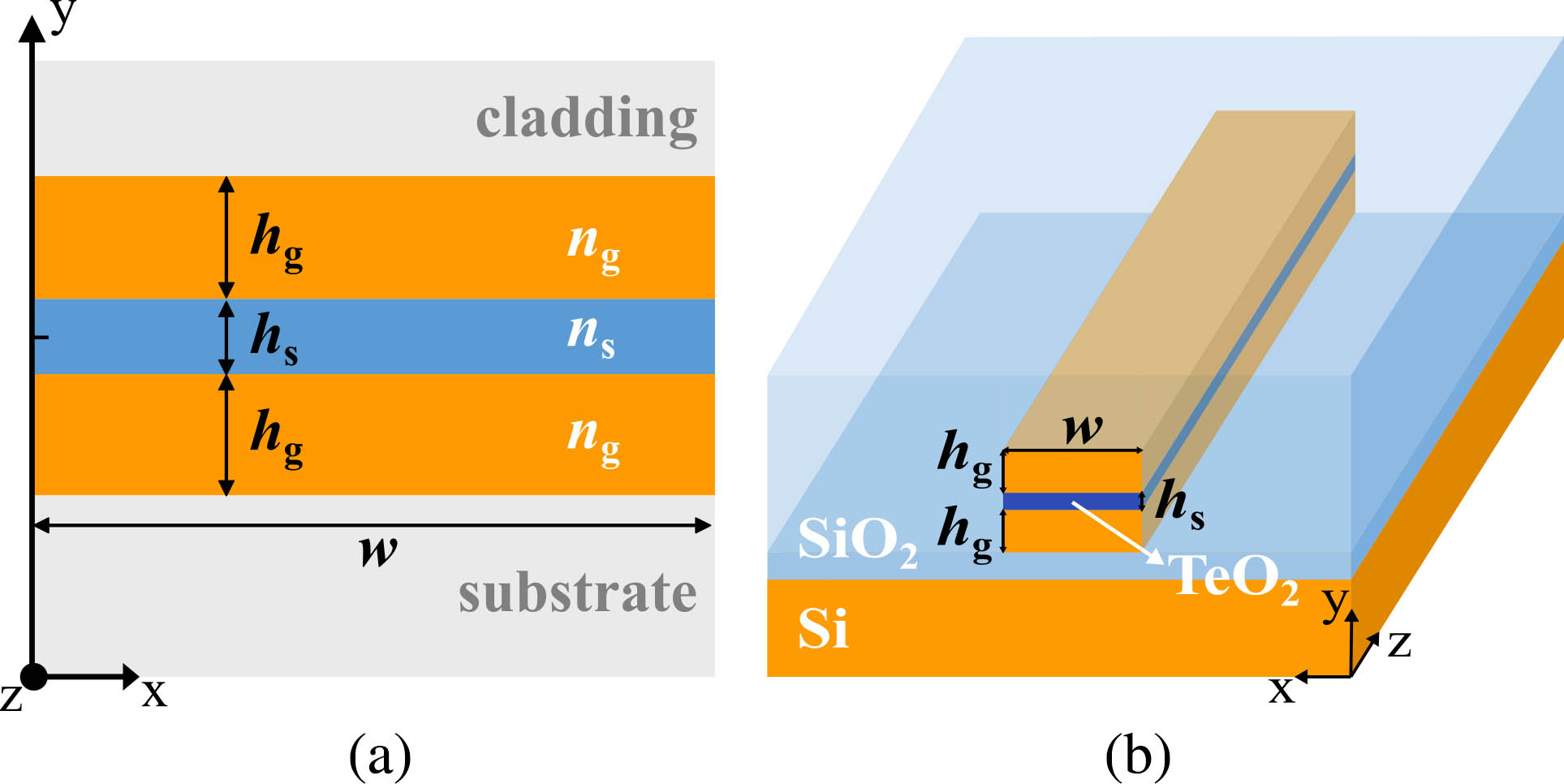
Set citation alerts for the article
Please enter your email address