
- Matter and Radiation at Extremes
- Vol. 6, Issue 5, 058401 (2021)
Abstract
I. INTRODUCTION
Organic–inorganic hybrid metal halides are promising materials for use in advanced optoelectronics applications owing to their unique structural and physical properties, such as soft lattices, adjustable bandgap, and high absorption coefficient.1–3 Dimensionality engineering at molecular levels has been demonstrated to be an effective route for increasing the diversity of their architectures and improving their stability as the optically active inorganic clusters are tailored by hydrophobic cations.4–6 Recently, low-dimensional (low-D) metal halides have drawn great interest for their outstanding properties, including broadband emission from self-trapped excitons (STEs), potential high emission efficiency, and large Stokes shift.7,8 Especially for the zero-dimensional (0D) metal halides, near-unity photoluminescence quantum yield (PLQY) has been achieved by optimizing compositions and local structures.9,10 Current research focuses mainly on chemical tailoring, such as cation engineering, which is limited by tunability and may introduce a variety of influencing factors.11–13 A systematic understanding of how structure tuning affects optical properties is still lacking, and there is therefore an urgent need for the development of advanced methods for regulation and in situ characterization.
It is well known that exciton emission properties depend strongly on lattice and electronic characteristics, such as structural distortions of polyhedral frameworks and the exciton binding energy.14–16 Thus, the exciton emission can be effectively modulated by controllably regulating the lattice and electronic features using chemical methods or external stimuli like temperature and pressure.17–19 The application of pressure is an effective and clean way to tune the structures and properties of functional materials.20–23 Using diamond anvil cells to apply high pressure, the bond distances and structural distortions of soft low-D metal halides can be effectively adjusted and their photophysical process and optical properties can be monitored in situ. There has recently been an increase in the number of studies focusing on pressure-enhanced and/or induced emission in low-D metal halides, although it is known that emission will be quenched at higher pressures. So far, no system has been found that can exhibit photoluminescence (PL) at pressures above 30 GPa.24,25 The microscopic origin of the enhancement also remains unclear.
In this work, we report pressure-induced, enhanced, and robust emission up to 80 GPa in a 0D hybrid metal halide (C9NH20)6Pb3Br12, hereinafter referred to as (bmpy)6[Pb3Br12] (where bmpy = 1-butyl-1-methylpyrrolidinium), which possesses linear [Pb3Br12]6− trimer clusters (face-sharing octahedra) isolated by organic cations.13,26 Using pressure regulation in combination with in situ structural and optical characterization, we have systematically investigated pressure-dependent photophysical properties. The pressure-induced emission (PIE) is attributed to the largely suppressed phonon-assisted nonradiative pathway. The exciton–phonon interaction is considerably reduced owing to the phonon hardening that occurs upon compression. Impressively, the emission of (bmpy)6[Pb3Br12] is still observable even at 80 GPa, which is the highest-recorded emission pressure for any kind of hybrid metal halide material. Such a robust emission is mainly due to the stiffness of the spring-like [Pb3Br12]6− trimers.
II. RESULTS AND DISCUSSION
To investigate the evolution of the emission properties of 0D (bmpy)6[Pb3Br12] under high pressure, in situ PL measurements were performed, as shown in Fig. 1. The emission is undetectable at ambient pressure, and a pressure-induced emission (PIE) centered at around 650 nm with a full width at half maximum (FWHM) of 175 nm can be observed at 1.6 GPa [inset in the left panel of Fig. 1(a)]. Such a broadband emission in this material originates from the excitons resulting from the strong electron–phonon coupling in the completely isolated individual [Pb3Br12]6− trimer clusters. As the pressure increases, the PL shows a remarkable enhancement and reaches a maximum at around 26 GPa. Upon further compression, the PL intensity gradually decreases with further compression [Fig. 1(a), right panel]. Figure 1(b) shows the integrated PL intensity of (bmpy)6[Pb3Br12] as a function of pressure. Strikingly, the emission can still be detected even at 80 GPa [inset in the right panel of Fig. 1(a)], a record pressure for emission in hybrid metal halides, indicating the extraordinary structural stability of this hybrid system. Such an unusually robust emission is likely due to the unique trimer clusters of this 0D metal halide, which we will elaborate on later. Besides, the fluorescence micrographs in Fig. 1(c) and in Fig. S1 in the
Figure 1.
Ultraviolet–visible (UV-vis) absorption spectroscopy was utilized to elucidate the bandgap evolution of (bmpy)6[Pb3Br12] under high pressure [Fig. 2(a) and Fig. S2 in the
Figure 2.Bandgap evolution of (bmpy)6[Pb3Br12] under high pressure. (a) UV-vis absorption spectra at high pressures. (b) Bandgap as a function of pressure. The insets show optical micrographs at selected pressures. (c) Bandgap evolution of (bmpy)6[Pb3Br12] compared with other hybrid metal halides, where the data are collected from the literature.
To better understand the pressure-induced, enhanced, and robust emission of this material, in situ x-ray diffraction (XRD) measurements were performed up to 80 GPa. Under ambient conditions, (bmpy)6[Pb3Br12] crystallizes in a trigonal structure with space group R
Figure 3.
The crystal structures under high pressure were refined using the Rietveld method (Fig. S6 in the
To the best of our knowledge, almost all the hybrid metal halides possess soft lattices, which impart a highly compressible structure whether they are 3D, 2D, 1D, or 0D in nature. When the pressure exceeds a certain threshold, the structure can no longer hold, resulting in amorphization. The emission will quench during crystalline structural damage. To date, no emission from these materials can survive above 30 GPa. As is summarized in Fig. 4(a) and Table I, the PL quenching pressure value of (bmpy)6[Pb3Br12] is as high as 80 GPa, far higher than that of any other low-D metal halide.14,24,35,39,42,43 Such a robust PL of (bmpy)6[Pb3Br12] could be attributable to its relatively high structural stability discussed above.
Figure 4.(a) PL emerging and quenching pressures of (bmpy)6[Pb3Br12] in comparison with those of other 0D and 1D metal halides. (b) Stokes shift and phonon energy as functions of pressure. (c) Schematic of pressure-induced emission from excitons. GS and ES indicate the ground state and excited state, respectively.
Chemical formula | Dimension | PIE pressure (GPa) | PL quenching pressure (GPa) | Reference |
---|---|---|---|---|
(bmpy)9[ZnBr4]2[Pb3Br11] | 0D | Ambient | 18.2 | |
(CH3NH3)3Bi2I9 | 0D | Ambient | 9.0 | |
Cs4PbBr6 | 0D | 3.01 | 18.23 | |
Cs3Bi2I9 | 0D | Ambient | 9.3 | |
C4N2H14PbBr4 | 1D | Ambient | 9.0 | |
C4N2H14PbBr4 | 1D | Ambient | 24.81 | |
C4N2H14SnBr4 | 1D | 2.06 | 20.02 | |
CH3(CH2)2NH3PbBr3 | 1D | Ambient | 7.3 | |
CsCu2I3 | 1D | Ambient | 16.0 | |
(BA)2PbI4 | 2D | Ambient | 10 | |
(BA)2PbI4 | 2D | Ambient | 12.6 | |
(PEA)2PbBr4 | 2D | Ambient | 15.6 | |
(PEA)2PbI4 | 2D | Ambient | 7.6 | |
(HA)2(GA)Pb2I7 | 2D | Ambient | 9.48 | |
(BA)2(MA)Pb2I7 | 2D | Ambient | 4.7 | |
(GA)(MA)2Pb2I7 | 2D | Ambient | 7.0 | |
(BA)4AgBiBr8 | 2D | 2.5 | 25.0 | |
(CH3NH3)PbCl3 | 3D | Ambient | 7.2 | |
(CH3NH3)PbBr3 | 3D | Ambient | 4.85 | |
(CH3NH3)PbBr3 | 3D | Ambient | 4.0 | |
(CH3NH3)PbI | 3D | Ambient | 2.7 | |
(CH3NH3)PbI1.2Br1.8 | 3D | Ambient | 1.6 | |
CsPb2Br5 | 3D | Ambient | 2.23 | |
Cs2AgBiCl6 | 3D | Ambient | 8.0 |
Table 1. Summary of PL emerging and quenching pressures for various metal halides.
To obtain the local bonding information under high pressure, in situ Raman spectra were collected, as shown in Fig. S7 in the
The strong exciton–phonon coupling gives rise to localized excitons and leads to the broadband emission in low-D metal halides. However, an overly strong exciton–phonon coupling would lead to overlap between the excited-state (ES) curve and the ground-state (GS) one, resulting in phonon-assisted nonradiative recombination of the excited electrons and holes.15 As has been reported, a relatively large Stokes shift exists in the low-D metal halides with face-sharing octahedra compared with the edge-sharing and corner-sharing compounds.12,13,56–58 As shown in Fig. 4(b), (bmpy)6[Pb3Br12] exhibits a large Stokes shift of 1.7 eV at 1.6 GPa, which decreases rapidly during compression. DFT calculations on electronic structures of (bmpy)6[Pb3Br12] reveal higher dispersive frontier orbitals under high pressures, which indicates reduced exciton localization (Fig. S3 in the
III. CONCLUSION
In summary, pressure-induced emission has been revealed in the 0D hybrid metal halide (bmpy)6[Pb3Br12], with PL emerging at around 1.6 GPa and surviving to a record high pressure of 80 GPa among all kinds of hybrid metal halides. Through comprehensive in situ high-pressure characterization and first-principles calculations, the pressure-induced emission has been demonstrated to be caused by suppression of the phonon-assisted nonradiative pathway. Lattice compression hardens the phonons and reduces the exciton–phonon interaction. The robust emission is attributed to the tough sublattice of optically active spring-like trimers [Pb3Br12]6−, indicated by the low compressibility with a relatively high bulk modulus K0 = 80.8 GPa and the slow bandgap decrease (12.1 meV/GPa). Our findings provide new insight into the design and optimization of trimers and oligomers in low-D metal halides with the aim of achieving high performance in optoelectronics applications.
SUPPLEMENTARY MATERIAL
ACKNOWLEDGMENTS
Acknowledgment. This work was supported by the National Nature Science Foundation of China (NSFC) (Grant Nos. U1930401 and 51527801). S.L. and B.M. are grateful for support from the National Science Foundation (Grant No. DMR-1709116). Portions of this work were performed at GeoSoilEnviroCARS (The University of Chicago, Sector 13), Advanced Photon Source (APS), Argonne National Laboratory. GeoSoilEnviroCARS is supported by the National Science Foundation – Earth Sciences (Grant No. EAR-1634415) and the Department of Energy – GeoSciences (Grant No. DE-FG02-94ER14466), and partially by COMPRES under NSF Cooperative Agreement No. EAR-1606856.
References
[1] Y.Chen, Y.Lei, Y.Li et al. Strain engineering and epitaxial stabilization of halide perovskites. Nature, 577, 209-215(2020).
[2] S.Bai, X.-K.Liu, W.Xu et al. Metal halide perovskites for light-emitting diodes. Nat. Mater., 20, 10-21(2020).
[3] M. M.Lee, T.Miyasaka, J.Teuscher et al. Efficient hybrid solar cells based on meso-superstructured organometal halide perovskites. Science, 338, 643-647(2012).
[4] J.-C.Blancon, W.Nie, H.Tsai et al. High-efficiency two-dimensional Ruddlesden–Popper perovskite solar cells. Nature, 536, 312-317(2016).
[5] Y.Tian, Z.Yuan, C.Zhou et al. One-dimensional organic lead halide perovskites with efficient bluish white-light emission. Nat. Commun., 8, 14051-14057(2017).
[6] S.Lee, L.Xu, C.Zhou et al. Recent advances in luminescent zero-dimensional organic metal halide hybrids. Adv. Opt. Mater., 2, 2001766(2020).
[7] Q.Fu, B.Huang, X.Tang et al. Recent progress on the long-term stability of perovskite solar cells. Adv. Sci., 5, 1700387(2018).
[8] H.Lin, Y.Tian, C.Zhou et al. Luminescent zero-dimensional organic metal halide hybrids with near-unity quantum efficiency. Chem. Sci., 9, 586-593(2018).
[9] H.Lin, Y.Tian, C.Zhou et al. Low-dimensional organometal halide perovskites. ACS Energy Lett., 3, 54-62(2018).
[10] M.Li, Z.Xia. Recent progress of zero-dimensional luminescent metal halides. Chem. Soc. Rev., 50, 2626-2662(2021).
[11] L.-K.Gong, J.-R.Li, Z.-F.Wu et al. Enhancing the phosphorescence of hybrid metal halides through molecular sensitization. J. Mater. Chem. C, 7, 9803-9807(2019).
[12] B.Febriansyah, D.Giovanni, C. S. D.Neo et al. Targeted synthesis of trimeric organic–bromoplumbate hybrids that display intrinsic, highly Stokes-shifted, broadband emission. Chem. Mater., 32, 4431-4441(2020).
[13] M.Li, L.Ning, J.Zhou et al. Broad-band emission in a zero-dimensional hybrid organic [PbBr6] trimer with intrinsic vacancies. J. Phys. Chem. Lett., 10, 1337-1341(2019).
[14] Z.Liu, S.Lu, Z.Ma et al. Pressure-induced emission of cesium lead halide perovskite nanocrystals. Nat. Commun., 9, 4506(2018).
[15] S.Li, J.Liu, J.Luo et al. Self-trapped excitons in all-inorganic halide perovskites: Fundamentals, status, and potential applications. J. Phys. Chem. Lett., 10, 1999-2007(2019).
[16] K.Bu, S.Guo, J.Li et al. Enhanced photocurrent of all-inorganic two-dimensional perovskite Cs2PbI2Cl2 via pressure-regulated excitonic features. J. Am. Chem. Soc., 143, 2545-2551(2021).
[17] M.Li, T.Liu, Y.Wang et al. Pressure responses of halide perovskites with various compositions, dimensionalities, and morphologies. Matter Radiat. Extremes, 5, 018201(2020).
[18] C.Pei, L.Wang. Recent progress on high-pressure and high-temperature studies of fullerenes and related materials. Matter Radiat. Extremes, 4, 028201(2019).
[19] C. S.Yoo. Chemistry under extreme conditions: Pressure evolution of chemical bonding and structure in dense solids. Matter Radiat. Extremes, 5, 018202(2020).
[20] Q.Hu, X.Lü, C.Stoumpos et al. Regulating off-centering distortion maximizes photoluminescence in halide perovskites. Natl. Sci. Rev.(2020).
[21] A.Navrotsky. Pressure-induced structural changes cause large enhancement of photoluminescence in halide perovskites: A quantitative relationship. Natl. Sci. Rev.(2021).
[22] H. K.Mao, W. L.Mao. Key problems of the four-dimensional earth system. Matter Radiat. Extremes, 5, 038102(2020).
[23] B.Chen, H.Gou, H. K.Mao et al. 2020—Transformative science in the pressure dimension. Matter Radiat. Extremes, 6, 013001(2020).
[24] Z.Ma, Y.Shi, D.Zhao et al. Pressure-induced emission (PIE) of one-dimensional organic tin bromide perovskites. J. Am. Chem. Soc., 141, 6504-6508(2019).
[25] K.Bu, S.Guo, Y.Zhao et al. Pressure-suppressed carrier trapping leads to enhanced emission in two-dimensional perovskite (HA)2(GA)Pb2I7. Angew. Chem., Int. Ed., 59, 17533-17539(2020).
[26] S.Lee, J.Neu, C.Zhou et al. Bulk assemblies of lead bromide trimer clusters with geometry-dependent photophysical properties. Chem. Mater., 32, 374-380(2020).
[27] B. J.Foley, D. L.Marlowe, K.Sun et al. Temperature dependent energy levels of methylammonium lead iodide perovskite. Appl. Phys. Lett., 106, 243904(2015).
[28] J.Gascon, F.Kapteijn, J. G.Santaclara et al. Understanding metal–organic frameworks for photocatalytic solar fuel production. CrystEngComm, 19, 4118-4125(2017).
[29] A.Jaffe, Y.Lin, W. L.Mao et al. Pressure-induced metallization of the halide perovskite (CH3NH3)PbI3. J. Am. Chem. Soc., 139, 4330-4333(2017).
[30] T.Cai, M.Que, H.Zhu et al. Pressure-induced phase transformation and band-gap engineering of formamidinium lead iodide perovskite nanocrystals. J. Phys. Chem. Lett., 9, 4199-4205(2018).
[31] P.Guo, L.Kong, G.Liu et al. Two regimes of bandgap red shift and partial ambient retention in pressure-treated two-dimensional perovskites. ACS Energy Lett., 2, 2518-2524(2017).
[32] X.Liu, X.Ma, Y.Yuan et al. Large band gap narrowing and prolonged carrier lifetime of (C4H9NH3)2PbI4 under high pressure. Adv. Sci., 6, 1900240(2019).
[33] Q.Li, W.Pan, Y.Wang et al. High-pressure band-gap engineering in lead-free Cs2AgBiBr6 double perovskite. Angew. Chem., Int. Ed., 129, 16185-16189(2017).
[34] A. S.Ahmad, X.Ren, X.Yan et al. Pressure-induced phase transition and band gap engineering in propylammonium lead bromide perovskite. J. Phys. Chem. C, 123, 15204-15208(2019).
[35] Z.Chen, M.Li, Q.Li et al. Pressure-engineered photoluminescence tuning in zero-dimensional lead bromide trimer clusters. Angew. Chem., Int. Ed., 60, 2583-2587(2020).
[36] Y.Lin, C.Liu, L.Zhang et al. Tuning optical and electronic properties in low-toxicity organic–inorganic hybrid (CH3NH3)3Bi2I9 under high pressure. J. Phys. Chem. Lett., 10, 1676-1683(2019).
[37] H. M.Rietveld. A profile refinement method for nuclear and magnetic structures. J. Appl. Crystallogr., 2, 65-71(1969).
[38] F.Birch. Finite elastic strain of cubic crystals. Phys. Rev., 71, 809-824(1947).
[39] Z.Chen, Q.Li, B.Yang et al. Pressure-induced remarkable enhancement of self-trapped exciton emission in one-dimensional CsCu2I3 with tetrahedral units. J. Am. Chem. Soc., 142, 1786-1791(2020).
[40] Y.Dai, Y.Gu, H.Liu et al. Pressure-induced blue-shifted and enhanced emission: A cooperative effect between aggregation-induced emission and energy-transfer suppression. J. Am. Chem. Soc., 142, 1153-1158(2020).
[41] D. C.Hooper, C.Kuppe, A. G.Mark et al. Strong rotational anisotropies affect nonlinear chiral metamaterials. Adv. Mater., 29, 1605110(2017).
[42] S.Guo, H.Luo, Y.Wang et al. Reaching 90% photoluminescence quantum yield in one-dimensional metal halide C4N2H14PbBr4 by pressure-suppressed nonradiative loss. J. Am. Chem. Soc., 142, 16001-16006(2020).
[43] F.Li, Z.Ma, L.Sui et al. Tunable color temperatures and emission enhancement in 1D halide perovskites under high pressure. Adv. Opt. Mater., 8, 2000713(2020).
[44] C.Liu, L.Wang, L.Zhang et al. Pressure-induced emission enhancement, band-gap narrowing, and metallization of halide perovskite Cs3Bi2I9. Angew. Chem., Int. Ed., 57, 11213-11217(2018).
[45] B.Liu, J.Yan, T.Yin et al. Pressure-engineered structural and optical properties of two-dimensional (C4H9NH3)2PbI4 perovskite exfoliated nm-thin flakes. J. Am. Chem. Soc., 141, 1235-1241(2019).
[46] K.Wang, L.Wu, L.Zhang et al. Pressure-induced broadband emission of 2D organic–inorganic hybrid perovskite. Adv. Sci., 6, 1801628(2019).
[47] C. K.Gan, S.Liu, S.Sun et al. Manipulating efficient light emission in two-dimensional perovskite crystals by pressure-induced anisotropic deformation. Sci. Adv., 5, eaav9445(2019).
[48] Y.Chen, R.Fu, L.Wang et al. Emission enhancement and bandgap retention of a two-dimensional mixed cation lead halide perovskite under high pressure. J. Mater. Chem. A, 7, 6357-6362(2019).
[49] Y.Fang, L.Wu, L.Zhang et al. Pressure‐induced emission (PIE) and phase transition of a two‐dimensional halide double perovskite (BA)4AgBiBr8 (BA=CH3(CH2)3NH3+). Angew. Chem., Int. Ed., 58, 15249(2019).
[50] T.Ishihara, K.Matsuishi, S.Onari et al. Optical properties and structural phase transitions of lead-halide based inorganic–organic 3D and 2D perovskite semiconductors under high pressure. Phys. Status Solidi B, 241, 3328-3333(2004).
[51] W. K.Chong, Y.Fang, T.Yin et al. High-pressure-induced comminution and recrystallization of CH3NH3PbBr3 nanocrystals as large thin nanoplates. Adv. Mater., 30, 1705017(2018).
[52] Y.Fang, S.Jiang, R.Li et al. Pressure-dependent polymorphism and band-gap tuning of methylammonium lead iodide perovskite. Angew. Chem., Int. Ed., 55, 6540-6544(2016).
[53] C. M.Beavers, A.Jaffe, Y.Lin et al. High-pressure single-crystal structures of 3D lead-halide hybrid perovskites and pressure effects on their electronic and optical properties. ACS Cent. Sci., 2, 201-209(2016).
[54] F.Li, Z.Ma, G.Qi et al. Structural stability and optical properties of two-dimensional perovskite-like CsPb2Br5 microplates in response to pressure. Nanoscale, 11, 820-825(2019).
[55] Y.Fang, L.Sui, L.Zhang et al. Tuning emission and electron–phonon coupling in lead-free halide double perovskite Cs2AgBiCl6 under pressure. ACS Energy Lett., 4, 2975-2982(2019).
[56] H.-H.Fang, M. R.Filip, M. E.Kamminga et al. Confinement effects in low-dimensional lead iodide perovskite hybrids. Chem. Mater., 28, 4554-4562(2016).
[57] H.Lin, M.Worku, C.Zhou et al. Blue emitting single crystalline assembly of metal halide clusters. J. Am. Chem. Soc., 140, 13181-13184(2018).
[58] X.Che, J. M.Hoffman, S.Sidhik et al. From 2D to 1D electronic dimensionality in halide perovskites with stepped and flat layers using propylammonium as a spacer. J. Am. Chem. Soc., 141, 10661-10676(2019).
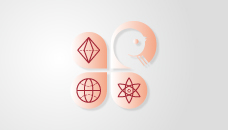
Set citation alerts for the article
Please enter your email address