Konrad Tschernig, Gabriel Martinez-Niconoff, Kurt Busch, Miguel A. Bandres, Armando Perez-Leija, "Topological protection of partially coherent light," Photonics Res. 10, 1223 (2022)

Search by keywords or author
- Photonics Research
- Vol. 10, Issue 5, 1223 (2022)
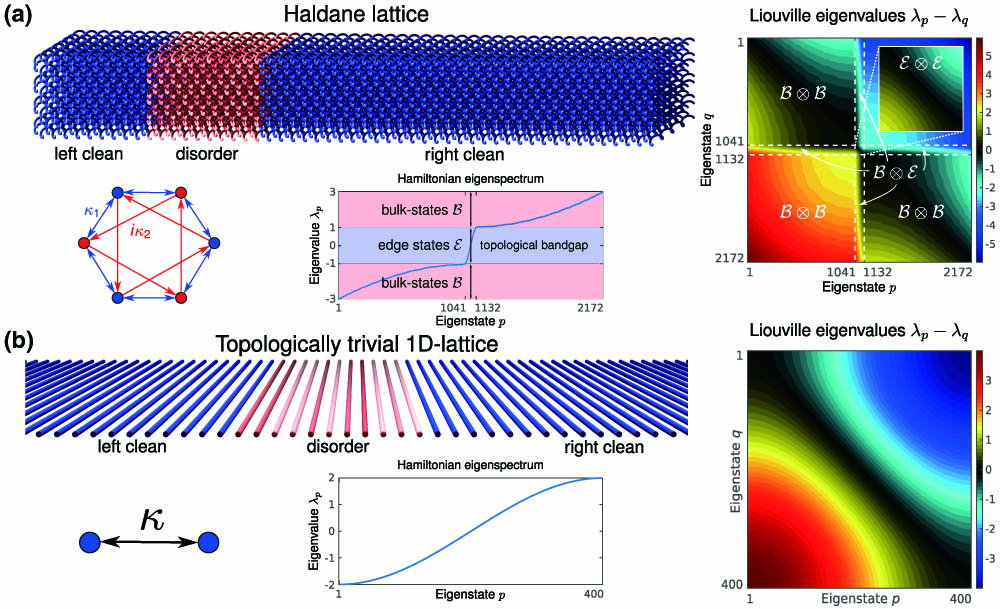
Fig. 1. Haldane lattice. (a) Photonic topological insulator implemented using a honeycomb lattice of helical waveguides with coupling coefficients as described in the hexagonal cell. For fully coherent light, the eigenspectrum exhibits two regions of bulk states and a gap crossed by the edge states E . For our analysis, we consider a lattice consisting of 11 hexagonal cells in the vertical direction and 90 in the horizontal, κ 1 = 1 , and κ 2 = 1 / 5 . (b) Trivial 1D lattice with couplings κ . In the absence of disorder, the discrete eigenspectrum exhibits a sinusoidal shape, and the corresponding Liouville eigenvalues are degenerated. In both cases, the Liouville eigenspectrum is shown in the right column for the disorder-free systems. The disordered regions (red waveguides) encompass 20 waveguides in the horizontal direction, the initial states are launched in the left clean regions, and they propagate to the right.
![Initial states. (a) and (b) The absolute values of the spatial |ρ^j,k| and spectral |ρ^p,q| coherences, respectively, for some examples of initial states ρ^α in the Haldane lattice. To improve the visibility of small components within the spectral edge-edge subspace, the insets in (b) depict |ρ^p,q|4. In (c) we show the spatial coherences |ρ^j,k| for the states obtained after removing all spectral bulk-bulk components. In all cases, we observe significant changes in the shape of the spatial coherences, and in the most extreme case (α=0), the removal of the spectral bulk components transforms a formerly incoherent state [leftmost panel in (a)] into a partially coherent one [leftmost panel in (c)].](/richHtml/prj/2022/10/5/05001223/img_002.jpg)
Fig. 2. Initial states. (a) and (b) The absolute values of the spatial | ρ ^ j , k | and spectral | ρ ^ p , q | coherences, respectively, for some examples of initial states ρ ^ α in the Haldane lattice. To improve the visibility of small components within the spectral edge-edge subspace, the insets in (b) depict | ρ ^ p , q | 4 . In (c) we show the spatial coherences | ρ ^ j , k | for the states obtained after removing all spectral bulk-bulk components. In all cases, we observe significant changes in the shape of the spatial coherences, and in the most extreme case ( α = 0 ) , the removal of the spectral bulk components transforms a formerly incoherent state [leftmost panel in (a)] into a partially coherent one [leftmost panel in (c)].
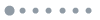
Fig. 3. Topological protection of coherent and partially coherent light states. (a) and (b) The initial and final intensities of the coherent ρ ^ c and the partially coherent ρ ^ i states, respectively. Both states are initialized with a Gaussian width σ = 6 , and the lattices exhibit a disorder strength Δ = 1 . After a propagation distance of z = 75 , both states reach the right side of the lattice. While the fully coherent excitation (a) freely passes through the disorder barrier, (b) the partially coherent state scatters into the bulk. Note that the cyan (green) spots indicate the intensity distribution of the initial (final) state, while the red spots show the disorder distribution. In (c)–(e) we show the degree of coherence μ 2 , fidelities, and transmittances as a function of the coherence parameter α for the states ρ ^ α in systems with disorder strength Δ = 1 .
![Transmittance and fidelity versus disorder strength Δ, spatial width σ, and degree of coherence of the initial states. (a) and (b) The transmittance T for the Haldane lattice and the 1D lattice versus the disorder strength Δ∈[0,2.5] and α, for initial states with a Gaussian width σ=6. Similarly, (c) and (d) show T as a function of α for initial excitations with spatial widths σ∈[0.1,6] in lattices with disorder strength Δ=1. (e) and (f) The fidelity F as a function of Δ and α for light states with spatial width σ=6.](/Images/icon/loading.gif)
Fig. 4. Transmittance and fidelity versus disorder strength Δ , spatial width σ , and degree of coherence of the initial states. (a) and (b) The transmittance T for the Haldane lattice and the 1D lattice versus the disorder strength Δ ∈ [ 0,2.5 ] and α , for initial states with a Gaussian width σ = 6 . Similarly, (c) and (d) show T as a function of α for initial excitations with spatial widths σ ∈ [ 0.1,6 ] in lattices with disorder strength Δ = 1 . (e) and (f) The fidelity F as a function of Δ and α for light states with spatial width σ = 6 .
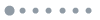
Fig. 5. The topological window of protection. To identify the topological window of protection, we considered a spectrally broad (spatially narrow) partially coherent state with σ = 0.1 as the initial state and propagate it through an ensemble of 5000 random Haldane lattices. In disorder-free systems, the spectral correlation map remains intact. As the disorder strength increases, e.g., (a) Δ = 0.5 , (b) Δ = 1 , and (c) Δ = 1.5 , the bandgap reduces, yielding to a reduction of the spectral window of protection as indicated by the dashed squares shown in the edge-edge subspace.
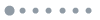
Fig. 6. Trivial Haldane lattice. Fully coherent state evolution in (a) the clean and (b) the disordered system. (c) Transmittance and (d) fidelity scans over disorder strength and coherence parameter.
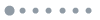
Fig. 7. Transmittance versus disorder strength and coherence parameter in the topological Haldane lattice for different disorder distributions. (a) Truncated Gaussian. (b) Gaussian. (c) Uniform. (d) Laplacian.
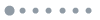
Fig. 8. Stationary defect modes in 1D arrays. (a) Topological defect mode of an SSH lattice. (b) Trivial defect mode of a regular lattice. (c), (d) Bound intensity and fidelity against disorder strength and coherence parameter in the SSH system and (e), (f) in the regular lattice.
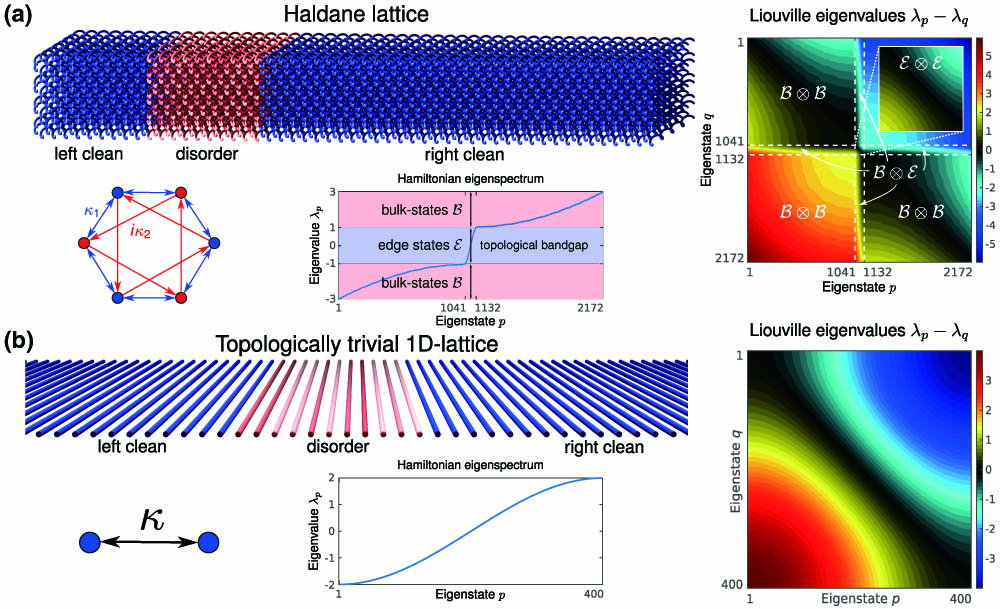
Set citation alerts for the article
Please enter your email address