Fig. 1. (a) Generic setup of a long-distance IFL [
79" target="_self" style="display: inline;">–9] transferring an optical frequency to a remote location. As an example, this setup is drawn based on fiber-optic interferometers and Faraday-rotator mirrors. The tracking filters are operated with a bandwidth higher than the propagation-delay-induced stabilization bandwidth [
8] and radio-frequency dividers are used for achieving unambiguous phase resolution. (b) Loop-back setup for implementing an OOL characterization via heterodyning the IFL input with the loop output. In applications, one can use (c) a repeater laser station (RLS) [
11] or (d) a manypoint extraction (MPE) [
38] for tapping off a stabilized optical frequency at the remote end and for simultaneous IFL performance assessment using the loop-back.
Fig. 2. Published state-of-the-art interferometric noise floor instabilities. The
Π-counted ADEV results from Ref. [
9] are indicated as possible
Λ-counted modADEV range assuming white-phase noise domination on short time scales.
Fig. 3. (a) Schematic setup for the OOL characterization of the optical frequency dissemination performance of the monolithic interferometer design. The fibers have been taped down to the optical table. In the case of S1 and S2 using single-mode fibers on the IFL, the polarization at the far end has been set to linear horizontal polarization in front of the retro-reflector. For the S2PM configuration, all fibers and components have been exchanged for polarization-maintaining variants. For the measurements, the free-space part of the setup has been covered with a cardboard box together with the photodiodes. (b) Top view of the assembled configuration showing the monolithic assembly, the non-polarizing beam splitter, the fused silica baseplate, and the fiber bench with fiber collimators. (c) Side view of the assembled configuration showing the position of the calibrated PT100 temperature sensor.
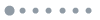
Fig. 4. Comparison of the instability of the OOL signal (red) to the estimated contributions of T variations (green) and p variations (blue) of the longest measurement runs in each of the three configurations. Temperature variations have been measured using a PT100 T probe close to the monolithic assembly as shown in Fig. 3(c). The light and dark gray curves display the instabilities of the IL signal and the servo AOM drive frequency, respectively. The latter can be interpreted as the frequency instability of the fiber connections. Air pressure data in (a) and (c) stem from a barometer placed in a neighboring laboratory in the same building at PTB. In (b), due to unavailability of data from the PTB barometer, air pressure data from the climate station of the Department of Hydrology and River Basin Management of Technical University Braunschweig have been used, which is ≈6 km apart. At times of overlapping operation, we have observed matching p instabilities of both barometers for averaging times τ>1000 s. This shows that the exact placement of the barometer is of minor importance for the assessment of the potential impact of p in the OOL characterization.
Fig. 5. (a) As an example, typical T and p variations in our lab. The data are the same as underlying Fig. 4(a). (b) Together with the T and p sensitivities from Eq. (9) one observes a close match of the measured OOL phase evolution with the estimated one for the S1 configuration.
Fig. 6. (a) Observed instability of the OOL signal (red curves with varying shade) and of the estimated
T (green curves with varying shade) and
p (blue curves with varying shade) variations for multiple runs in the S2PM configuration. For comparison the instabilities published by Akatsuka
et al. [
36] and Cantin
et al. [
37] are included. The gray line shows the instability of the IL signal when the PD signal is replaced by an electronic signal. (b) Typical OOL phase noise densities for configuration S2PM and for modified setups without using tracking filters (TFs) and using a field-programmable gated array-based PLL [
59]. The prominent phase noise peaks around 1 kHz are the servo bumps of the IFL stabilization. The lower phase noise reduction achieved for the blue trace compared to the orange between 10 kHz and 1 MHz is due to the tracking filter. The phase noise feature in the blue curve at
≈300 kHz is the servo bump of the tracking filter PLL.
Fig. 7. (a) Π and Λ-averaged OOL frequency offsets over the different measurement runs. The statistical uncertainty of the offsets is estimated by the overlapping ADEV and modADEV, respectively, at an averaging time of τ=Td/4 (Td, duration of the measurement run). (b) Residuals of the OOL frequency offsets after accounting for the estimated phase contribution of the measured T and p variations. The light and dark gray curves display the instabilities of the IL stabilization and the servo AOM drive frequency, respectively. The latter can be regarded as the free-running fiber noise of the IFL fibers.
Fig. 8. Temperature sensitivities γT per optical path length L for different optical glasses from the vendors Schott, Ohara, Hoya, CDGM, and Sumita in comparison to γT,OOL(S1)/LS1, γT,OOL(S2)/LS2, and γT(air)/Lair. Shown are the four least temperature-sensitive glasses of each manufacturer. Considering all glasses listed in the respective catalogues, the values spread over a range from ≈26.5 fs K−1 mm−1 to ≈100 fs K−1 mm−1.
Ref. | Configuration | IFL length | T stabilization | Estimated correlation , , and | Estimated magnitude | Type of counting & ADEV | Accuracy interferometric noise floor () |
---|
[8] | Free-space on Al breadboard | 76 km | Passive | Partial | Large | | | [9] | Fiber, optimized for exploiting correlations of , , and | Short-cut | Passive | Partial–high | Low–moderate | , ADEV | 3.5 | [12] | Fiber, IL, and remote reference arm separately two-layer T shielded (inside Al housing in wooden box), same building/climatization as in Ref. [9] | Short-cut | Passive | Partial | Moderate–large | , modADEV | – | [30] | Fiber, IL, and remote reference arm and connection in same housing | 43 km | Passive | High | Low | , modADEV | | [41] | Fiber, IL, and remote reference arm and connection in same T stabilized housing | Short-cut | Active | High | Low | , modADEV | – | [36] | Multi-branch distribution, compact planar light wave circuit, two-branch difference | Short-cut | Passive | High | Low | modADEV | – | [37] | Multi-branch distribution, compact free-space, two-branch difference | Short-cut | Passive | Partial | Low | , modADEV | – |
|
Table 1. Summary of Literature Interferometric Noise Floor Results Together with Disclosed Configuration Details and a Qualitative Classificationa
Material | Element | | [] | [] | [] | [] |
---|
N-BK7 [50] | NBS | 1.50075 | 0.85310 | 7.10 | [51] | 46.5 | Corning 7980 [52] | PBS | 1.44408 | 9.4893 | 0.52 | [51] | 35.9 | UV fused silica [53] | Base | | | 0.55 | | 37.2 | Quartz [54] | | 1.52778 (o) | −5.6 (o) | 12.4 | 1.03 (o) | at | | | 1.53629 (e) | −6.7 (e) | | 1.075 (e) | 589 nm | Stainless steel | Bench | | | 10 | | 160 | Air [55,56] | | 1.00027 | −0.91049 | | 265.19 | |
|
Table 2. Materials Properties Used for the Calculation of the Environmental Sensitivities (Operating Conditions: λ=1542 nm, T=20°C, p=1004.04 hPa, 0% Relative Humidity, 450 ppm CO2 Concentration)a
Quantity | Path | [fs/K] | [as/hPa] |
---|
| PBS–HR–PBS | 0.18 | | | PBS–NBS–fiber end | 1.68 | 31.85 | | PBS–NBS–PR | 0.33 | 3.98 |
|
Table 3. Environmental Sensitivity γχ of different Paths at Operation Wavelength λ=1542 nm