
- Photonics Research
- Vol. 10, Issue 2, 289 (2022)
Abstract
1. INTRODUCTION
Terahertz (THz) radiation, ranging from 0.1 to 10 THz, can easily penetrate diverse non-polarity, non-metallic materials without ionization, but can be strongly absorbed by liquids and other states of water molecules. Various THz spectroscopic and imaging methods have been developed with different configurations to accommodate the properties of THz waves and scenarios. In particular, the approaches of THz refractive index (RI) measurements have been validated in numerous aspects of applications, e.g., non-destructive defect testing of aircraft composite materials from mechanical or heat damage [1], complementary medical diagnosis of melanoma and brain gliomas [2,3], and pigment identification in cultural relic restoration [4].
THz time-domain spectroscopy (THz-TDS), as the cornerstone of THz imaging and a dominant commercial measurement technique, can measure the sample’s RI curve versus wavelength by Fourier analysis of a complete THz pulse waveform. Two-dimensional (2D) RI distributions are mainly obtained by time-consuming raster scanning. The transmissive configuration is available to collect the mean axial RI distribution of the samples [5], which have low absorption and
In addition, THz tomography is available to retrieve the three-dimensional RI distribution of the sample with the assumption of a slow RI change in the sample and its surrounding medium. One category of THz tomography, denoted as THz computed tomography (CT), is based on a geometrical straight-line model of the radiation propagation that neglects the diffraction effects and Fresnel losses [11,12]. The other major category, known as THz diffraction tomography (DT), is based on the weak scattering approximation that accounts for the diffraction effect [13–15]. Therefore, the accuracy of RI measurements by THz DT is usually higher than by THz CT, especially for samples with fine structure. The current approaches are very difficult in measuring full-field RI distributions dynamically in the THz band, particularly for liquid samples.
Sign up for Photonics Research TOC. Get the latest issue of Photonics Research delivered right to you!Sign up now
In the visible-light domain, TIR digital holography (TIR-DH) is proposed to achieve full-field measurements of RI distributions. In the initial configuration, several on-axis holograms are recorded by the interference between the fixed-step phase-shifted reference beam and the TIR beam [16]. It is validated that the changes of the RPV are greater when the prism is irradiated by the
In the THz domain, with the development of array detectors and CW coherent sources, the variations of visible-light DH, which are categorized as THz DH (TDH), are proliferating in THz lensless full-field phase imaging. In-line TDH achieves sub-wavelength lateral resolution for small-sized objects [22]. However, iterative phase retrieval algorithms are required to suppress the twin image [23–25]. Both the size restriction and time-consuming reconstruction in this layout can be relieved by using the transport of intensity equation [26]. The twin image can also be minimized by phase-shifting TDH; nevertheless, the lateral resolution declines because of the extended recording distance for the beam splitter (BS) [27]. Off-axis Fresnel TDH has both transmissive and reflective geometries for different usage scenarios [28–32]. Spatial Fourier filtering, autofocusing, angular spectrum integral, and phase unwrapping are adopted in sequence for the numerical reconstruction. The second and third reconstruction steps can be replaced by adopting a single inverse Fourier transform in lensless Fourier-transform TDH. In this triangular interferometric layout, the reference beam is brought to focus at the object plane, and is equidistant from the recording plane. In addition, the environmental disturbances can be suppressed via the common-path self-referencing configuration [33].
Nevertheless, TDH has not been used for measuring RI distributions nor has TIR-DH been realized in the THz band. The main difficulty for these measurements might be the vertical orientation adjustment of the visually opaque HRFZ-Si ATRP, which is irradiated by the invisible THz beam. The pitching angle of the prism as well as the effective field of view (FOV) is difficult to measure. On the other hand, the current theoretical models in TIR-DH assume that the bottom of the ATRP is parallel to the horizontal plane [16–21].
In this study, we propose the total internal reflection terahertz digital holography (TIR-TDH) and apply it for measuring THz dynamic full-field RI distributions for both solid and liquid samples. A theoretical model is established based on a vertically tilted ATRP irradiated by a
2. REFRACTIVE INDEX MEASUREMENT MODEL
The principle of TIR-TDH based on a vertically tilted ATRP is illustrated in Fig. 1. To avoid a considerable RI measurement error, the pitching angle of the ATRP is considered in the following deductions.
Figure 1.Schematic illustration of TIR-TDH when the ATRP is vertically tilted.
It is shown in Fig. 1 that the left side of the ATRP is illuminated by a monochromatic
When the illumination beam has a circular profile with a diameter of
We assume that
The emergent beam propagates through the right side of the prism, thereafter interferes with the reference beam, and generates an off-axis Fresnel hologram [36]:
The hologram is spatially filtered to eliminate the twin image and thereafter is tilt-corrected based on transformation and rescaling of the coordinates to avoid anamorphism [37]. The complex amplitude at the recording plane thereafter propagates backward to the object plane using the angular spectrum integral [36].
In the experiments, the retrieved phase distribution at the object plane is constituted by the RPV and an additional phase disturbance induced by the experimental setup and the surrounding environment. The phase disturbance can be suppressed via the two-step phase subtraction method [17], assuming the disturbance is invariant during data acquisition. The RPV can be calculated by [17]
3. RESULTS
A. Experimental Setup
A schematic diagram of the experimental setup is depicted in Fig. 2. An infrared-pumped laser (295-FIRL-HP, Edinburgh Instruments) emitted a CW THz beam at a wavelength of 118.83 μm (2.52 THz) with a maximum output power of up to 500 mW. The diameter and the divergence angle of the beam were
Figure 2.Sketch of the actual TIR-TDH system. HWP, half-wave plate; PMs, parabolic mirrors; BS, beam splitter; M, mirror; ATRP, attenuated total reflection prism.
It is noted that alignment is necessary before inserting the ATRP in the layout. According to the deduction listed in Appendix A, when both the illumination beam and the reference beam are parallel with the optical table, the “
B. Full-Field Refractive Index Measurement
To verify the effectiveness of the full-field 2D RI measurements, a solid droplet of soy wax was adopted. This material consists of a mixture of several higher order alkanes and has a lower phase transition curve than paraffin wax. The sample’s RI was 1.495 at 2.52 THz, measured by a transmissive THz-TDS system (TeraPulse 4000, Teraview), in which the melted soy wax was congealed and pressed onto a solid wafer with a thickness of 2.28 mm.
At the beginning, 500 frames of the background hologram were recorded without the sample, and the accumulated hologram via Gaussian fitting [23] is denoted as Ibg. The soy wax was heated to
Figure 3.RI measurement results of a soy wax droplet. (a) Photograph of the sample. (b) Hologram
In contrast to transmissive geometries, the TIR-TDH system is also available to measure the 2D RI distribution of liquids. A droplet of distilled water, as shown in Fig. 4(a), was adopted as another sample. The acquisition time of the object hologram was
Figure 4.RI measurement results of a distilled water droplet. (a) Photograph of sample. (b) Hologram
C. Dynamic Refractive Index Measurement
The dynamic RI distribution measurement was also performed to monitor the evaporation process of a droplet constituted by 25% water and 75% ethanol. The RI distribution of the ethanol solution droplet is uniform in the initial state. However, the volatilization rates of ethanol and water are quite different, and the volatilization situations at different surface regions between the droplet and air are not identical, both of which lead to the dynamic diffusion of ethanol inside the droplet. Therefore, the RI distribution at the droplet–prism interface should be anisotropic and dynamic. The RI value is close to that of air in the region where the droplet volatilizes. The remaining droplet is mostly water and has an RI close to that of water. Therefore, a volatile ethanol droplet is an appropriate test sample to validate the feasibility of measuring 2D RI distribution dynamically.
In this experiment, every 10 successive frames were accumulated to form a hologram without Gaussian fitting, since 10 frames are statistically insufficient to extract the expectancy of the intensity from the Gaussian statistics. The interval of the temporal adjacent holograms was five frames. Therefore, the video rate of the RI distribution measurement was 10 Hz (see
Figures 5(a) and 5(b) illustrate nine photographs with a fixed time interval of 1.5 s and the corresponding RI distributions at 2.52 THz. The area of the droplet decreased by 78% from 10 to 22 s. As the thickness of the droplet became thinner during evaporation, the boundary ring became more obvious. It is illustrated in Fig. 5(b) that a
Figure 5.THz RI measurement of an evaporating 75% ethanol droplet. (a) Photographs at different moments. (b) Corresponding 2D RI distributions at 2.52 THz. (c) Changes in the average RI values over time in different regions illustrated in (b). (d) Varied RI data extracted from the white line in (b).
4. DISCUSSION AND CONCLUSION
In summary, we proposed TIR-TDH, which enables dynamic full-field measurement of the 2D RI distribution at the THz band. Previously, it was not possible to measure the dynamic full-field RI distribution of liquid samples in the THz domain, with only some transmissive solid samples of fixed thicknesses that could be measured. The availability of full-field and dynamic RI measurements was experimentally validated by the TIR-TDH system using different solid and liquid samples. The proposed method greatly expands the range of samples and provides unprecedented insight into measuring static and dynamic THz RI distributions. TIR-TDH has diverse potential usage scenarios, e.g., using RI as an indicator to distinguish between cancerous and normal tissues [40], observation of the RI variations of gaseous and liquid turbulence under microscope conditions, or characterizing different phase change materials for manufacturing THz modulators and diffraction optical components.
In the case of a droplet of distilled water as the sample in our setup, the RI error achieves 0.13%, 0.26%, 1.37%, and 2.85% when the pitching angle is 0.5°, 1°, 5°, and 10°, respectively. In addition, the ratio of D'/D decreases monotonously from 3.4 to 2.0 when the pitching angle changes from
In addition, in the reported THz TIR imaging and RI measurement geometries, the sample was mainly illuminated by a convergent beam. In our configuration, samples are irradiated by a parallel beam with a fixed angle of incidence, thus improving the accuracy of RI measurement. The environmental disturbance can be suppressed by applying the two-step phase subtraction method.
So far, the interval of temporal adjacent RI distributions is 0.1 s, while the data acquisition speed, i.e., the chopping frequency of the pyroelectric detector, is 50 Hz. The bottleneck of the time resolution is mainly the low SNR of the single frame hologram. This circumstance can be easily improved by using microbolometers instead of pyroelectric detectors. The experimental setup is stable during holographic data acquisition. Nevertheless, its long-term stability is challenged. A bottle of 40 L mixture gas (7%
APPENDIX A: ESTIMATING THE PITCHING ANGLE FROM THE OFF-AXIS HOLOGRAM
It is shown in Fig.
Figure 6.(a) Orientation of the object beam and reference beam in the coordinates of
According to Eq. (
The spatial frequency in the vertical direction is the reciprocal of the fringe period:
It can be deduced from Eq. (
References
[1] C. D. Stoik, M. J. Bohn, J. L. Blackshire. Nondestructive evaluation of aircraft composites using transmissive terahertz time domain spectroscopy. Opt. Express, 16, 17039-17051(2008).
[2] D. Li, Z. Yang, A. Fu, T. Chen, L. Chen, M. Tang, H. Zhang, N. Mu, S. Wang, G. Liang, H. Wang. Detecting melanoma with a terahertz spectroscopy imaging technique. Spectrochim. Acta A, 234, 118229(2020).
[3] Y. B. Ji, S. J. Oh, S. Kang, J. Heo, S. Kim, Y. Choi, S. Song, H. Y. Son, S. H. Kim, J. H. Lee, S. J. Haam, Y. M. Huh, J. H. Chang, C. Joo, J. Suh. Terahertz reflectometry imaging for low and high grade gliomas. Sci. Rep., 6, 36040(2016).
[4] J. B. Jackson, M. Mourou, J. F. Whitaker, I. N. Duling, S. L. Williamson, M. Menu, G. A. Mourou. Terahertz imaging for non-destructive evaluation of mural painting. Opt. Commun., 281, 524-532(2008).
[5] E. Jung, M. Lim, K. Moon, Y. Do, S. Lee, H. Han, H. J. Choi, K. Cho, K. Kim. Terahertz pulse imaging of micro-metastatic lymph nodes in early-stage cervical cancer patients. J. Opt. Soc. Korea, 15, 155-160(2011).
[6] S. Fan, E. P. J. Parrott, B. S. Y. Ung, E. Pickwell-MacPherson. Calibration method to improve the accuracy of THz imaging and spectroscopy in reflection geometry. Photon. Res., 4, A29-A35(2016).
[7] K. Shiraga, Y. Ogawa, T. Suzuki, N. Kondo, A. Irisawa, M. Imamura. Characterization of dielectric responses of human cancer cells in the terahertz region. J. Infrared Millim. Terahertz Waves, 35, 493-502(2014).
[8] M. Born, E. Wolf. Principles of Optics: Electromagnetic Theory of Propagation, Interference and Diffraction of Light(2013).
[9] A. Roggenbuck, H. Schmitz, A. Deninger, I. C. Mayorga, J. Hemberger, R. Güsten, M. Grüninger. Coherent broadband continuous-wave terahertz spectroscopy on solid-state samples. New J. Phys., 12, 043017(2010).
[10] S. Dülme, N. Schrinski, B. Khani, P. Lu, V. Rymanov, A. Stöhr, C. Brenner, M. R. Hofmann. Compact optoelectronic THz frequency domain spectroscopy system for refractive index determination based on Fabry-Perot effect. First International Workshop on Mobile Terahertz Systems (IWMTS), 1-5(2018).
[11] L. Chen, Y. Wang, D. Xu, Y. Ren, Y. He, C. Li, C. Zhang, L. Tang, C. Yan, J. Yao. Terahertz computed tomography of high-refractive index objects based on refractive index matching. IEEE Photon. J., 10, 5900813(2018).
[12] D. Wang, R. Ning, G. Li, J. Zhao, Y. Wang, L. Rong. 3D image reconstruction of terahertz computed tomography at sparse angles by total variation minimization. Appl. Opt., 61, B1-B7(2021).
[13] S. Wang, B. Ferguson, D. Abbott, X. Zhang. T-ray imaging and tomography. J. Biol. Phys., 29, 247-256(2003).
[14] M. Suga, Y. Sasaki, T. Sasahara, T. Yuasa, C. Otani. THz phase-contrast computed tomography based on Mach-Zehnder interferometer using continuous wave source: proof of the concept. Opt. Express, 21, 25389-25402(2013).
[15] D. Wang, X. Jin, J. Zhao, Y. Wang, L. Rong, J. J. Healy. Continuous-wave terahertz diffraction tomography for measuring three-dimensional refractive index maps. Chin. Opt. Lett., 19, 123701(2021).
[16] Z. Jian, P. Hsieh, H. Hsieh, H. Chen, D. Su. A method for measuring two-dimensional refractive index distribution with the total internal reflection of
[17] Y. Zhou, H. Zou, L. Zhong, J. Li, B. Li, J. Tian, X. Lu. Dynamic refractive index distribution measurement of dynamic process by combining dual-channel simultaneous phase-shifting interferometry and total internal reflection. Sci. Rep., 8, 15231(2018).
[18] W. M. Ash, M. K. Kim. Digital holography of total internal reflection. Opt. Express, 16, 9811-9820(2008).
[19] J. Zhang, J. Di, Y. Li, T. Xi, J. Zhao. Dynamical measurement of refractive index distribution using digital holographic interferometry based on total internal reflection. Opt. Express, 23, 27328-27334(2015).
[20] A. Calabuig, M. Matrecano, M. Paturzo, P. Ferraro. Common-path configuration in total internal reflection digital holography microscopy. Opt. Lett., 39, 2471-2474(2014).
[21] J. Zhang, C. Ma, S. Dai, J. Di, Y. Li, T. Xi, J. Zhao. Transmission and total internal reflection integrated digital holographic microscopy. Opt. Lett., 41, 3844-3847(2016).
[22] Z. Li, Q. Yan, Y. Qin, W. Kong, G. Li, M. Zou, D. Wang, Z. You, X. Zhou. Sparsity-based continuous wave terahertz lens-free on-chip holography with sub-wavelength resolution. Opt. Express, 27, 702-713(2019).
[23] L. Rong, T. Latychevskaia, D. Wang, X. Zhou, H. Huang, Z. Li, Y. Wang. Terahertz in-line digital holography of dragonfly hindwing: amplitude and phase reconstruction at enhanced resolution by extrapolation. Opt. Express, 22, 17236-17245(2014).
[24] L. Rong, T. Latychevskaia, C. Chen, D. Wang, Z. Yu, X. Zhou, Z. Li, H. Huang, Y. Wang, Z. Zhou. Terahertz in-line digital holography of human hepatocellular carcinoma tissue. Sci. Rep., 5, 8445(2015).
[25] Z. Li, R. Zou, W. Kong, X. Wang, Q. Deng, Q. Yan, Y. Qin, W. Wu, X. Zhou. Terahertz synthetic aperture in-line holography with intensity correction and sparsity autofocusing reconstruction. Photon. Res., 7, 1391-1399(2019).
[26] L. Rong, S. Wang, D. Wang, F. Tan, Y. Zhang, J. Zhao, Y. Wang. Transport of intensity equation-based terahertz lensless full-field phase imaging. Opt. Lett., 46, 5846-5849(2021).
[27] M. Yamagiwa, T. Minamikawa, F. Minamiji, T. Mizuno, Y. Tokizane, R. Oe, H. Koresawa, Y. Mizutani, T. Iwata, H. Yamamoto, T. Yasui. Visualization of internal structure and internal stress in visibly opaque objects using full-field phase-shifting terahertz digital holography. Opt. Express, 27, 33854-33868(2019).
[28] E. Hack, P. Zolliker. Terahertz holography for imaging amplitude and phase objects. Opt. Express, 22, 16079-16086(2014).
[29] M. Locatelli, M. Ravaro, S. Bartalini, L. Consolino, M. S. Vitiello, R. Cicchi, F. Pavone, P. De Natale. Real-time terahertz digital holography with a quantum cascade laser. Sci. Rep., 5, 13566(2015).
[30] P. Zolliker, E. Hack. THz holography in reflection using a high resolution microbolometer array. Opt. Express, 23, 10957-10967(2015).
[31] L. Valzania, P. Zolliker, E. Hack. Topography of hidden objects using THz digital holography with multi-beam interferences. Opt. Express, 25, 11038-11047(2017).
[32] D. Wang, Y. Zhao, L. Rong, M. Wan, X. Shi, Y. Wang, J. T. Sheridan. Expanding the field-of-view and profile measurement of covered objects in continuous-wave terahertz reflective digital holography. Opt. Eng., 58, 023111(2019).
[33] D. Wang, Y. Zhang, L. Rong, D. Ma, J. Zhao, Y. Wang. Continuous-wave terahertz self-referencing digital holography based on Fresnel’s mirrors. Opt. Lett., 45, 913-916(2020).
[34] K. Artmann. Berechnung der Seitenversetzung des totalreflektierten Strahles. Ann. Phys., 437, 87-102(1948).
[35] H. Wang, Y. Wang, D. Xu, L. Wu, C. Yan, D. Yan, L. Tang, Y. He, H. Feng, J. Yao. High-sensitivity attenuated total internal reflection continuous-wave terahertz imaging. J. Phys. D, 50, 375103(2017).
[36] J. W. Goodman. Introduction to Fourier Optics(2005).
[37] Y. Kim, G. Kim, H. Ryu, H. Chu, C. Hwang. Exact light propagation between rotated planes using non-uniform sampling and angular spectrum method. Opt. Commun., 344, 1-6(2015).
[38] J. Dai, J. Zhang, W. Zhang, D. Grischkowsky. Terahertz time-domain spectroscopy characterization of the far-infrared absorption and index of refraction of high-resistivity, float-zone silicon. J. Opt. Soc. Am. B, 21, 1379-1386(2004).
[39] P. Yeh. Optical Waves in Layered Media(1988).
[40] N. Vohra, T. Bowman, K. Bailey, M. El-Shenawee. Terahertz imaging and characterization protocol for freshly excised breast cancer tumors. J. Vis. Exp., 158, e61007(2020).
[41] Y. Gan, B. Mirzaei, S. van der Poel, J. R. G. Silva, M. Finkel, M. Eggens, M. Ridder, A. Khalatpour, Q. Hu, F. van der Tak, J. Gao. 3.9 THz spatial filter based on a back-to-back Si-lens system. Opt. Express, 28, 32693-32708(2020).
[42] T.-C. Poon, J.-P. Liu. Introduction to Modern Digital Holography with MATLAB(2014).
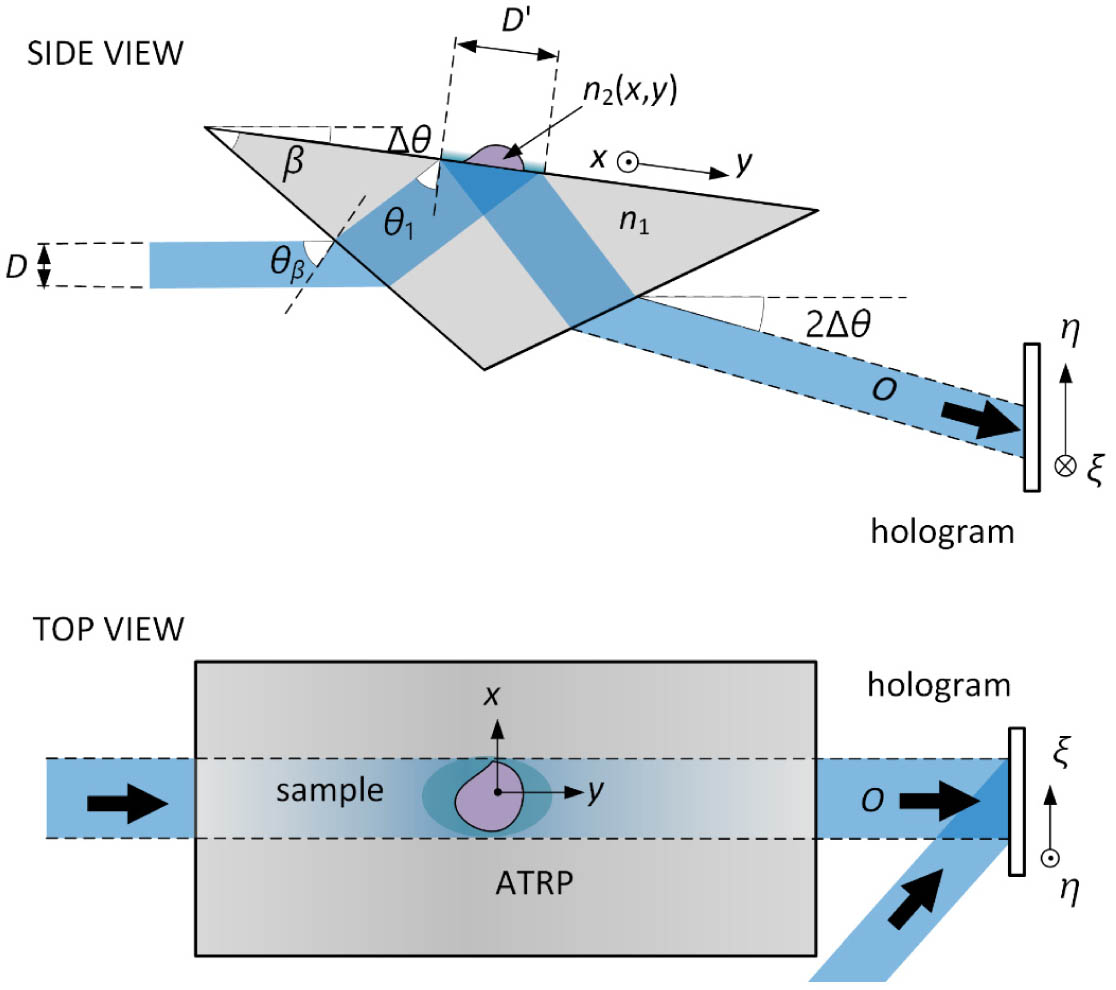
Set citation alerts for the article
Please enter your email address