Abstract
We propose and demonstrate a laser-diode-pumped, maglev rotating Nd:YAG disk laser. The disk of the laser crystal is attached to a maglev pyrolytic graphite disk and is rotated by compressed gas. In this rotating disk laser, the detrimental thermal effects are alleviated and the laser can be operated in the single transverse electromagnetic mode with high brightness. In our proof-of-concept experiment, we achieve a 17.7 mW laser output at 447 mW of absorbed pump power and a rotation frequency.The waste heat produced in any solid-state laser medium is an unavoidable problem that can cause thermally induced birefringence[1], thermal stress[2] and thermal lensing[3]. It imposes fundamental limitations on the brightness and average power scaling of solid-state lasers[4]. High single-mode power and a high laser brightness factor are required in many applications, such as nonlinear optics, optical communication in space, and precision laser machining. In order to significantly increase the single-mode output power in solid-state lasers, the concept of using fiber-coupled laser diodes to pump a rotating disk laser was invented by Basu and Byer in 1987[5]. In a rotating disk laser, the pump beam is focused on a small spot on the disk away from the center, and the rotation of the disk continuously removes the heated material from the pumped area and brings in cold material to be pumped. If the pumping spot, as well as lasing area pair, is moved at a velocity greater than the time scales associated with the heat flow but slower than those associated with lasing, it is thus possible to avoid detrimental thermal effects while maintaining the lasing performance. The first experimental result on a diode-pumped rotating Nd:glass disk laser was reported by Korn et al. in 1991[6]. From then on, many other rotary disk lasers with continuous-wave (CW) or -switching operations have been reported[7–10]. However, these existing rotary disk lasers must have an electric motor to rotate the gain medium disk, which makes the laser system complicated and unstable.
Fortunately, there is another possible way to make the disk of a laser gain medium rotate. As is known[11,12], some of the strongest diamagnetic materials, such as graphite and bismuth, can be levitated above NdFeB permanent magnets at room temperature. Further, a magnetically levitating diamagnetic material can be effectively manipulated by a weak force. When exposed to the irradiation of a laser beam, the change of magnetic susceptibilities due to the local temperature rise in the levitating pyrolytic graphite (PG) disk activates the rotation of itself[13]. Thus, when a disk of laser gain medium with the proper weight is attached to the upper surface of the diamagnetic material, both the laser crystal and diamagnetic material can be levitated and will rotate in the presence of laser beam irradiation. Further, by incorporating the disk of laser gain medium into a laser resonator and by utilizing the unabsorbed pump light transmitting through the gain medium to induce the rotation of the PG plate levitated above the permanent magnets, a maglev rotating disk laser would be feasible.
Based on this consideration, in this study, we presented an initial investigation on a maglev rotating disk laser. In the investigation, a thin disk from an Nd:YAG crystal was used as the gain medium and attached to a PG plate levitated above permanent magnets. Owing to the fact that this compound was too heavy to be rotated by the rotational kinetic energy activated by the unabsorbed pump power, the compressed nitrogen ejected by two nozzles was used to drive the rotation of the compound. With this arrangement, we realized a maglev rotating Nd:YAG laser, and achieved a single-mode output of 17.7 mW at 447 mW of absorbed pumped power and a rotation frequency of .
Sign up for Chinese Optics Letters TOC. Get the latest issue of Chinese Optics Letters delivered right to you!Sign up now
The experiment setup of this study is shown in Fig. 1. It consisted of a laser-diode (LD) end-pumping laser system and a PG maglev system. In the laser, pump source was a fiber-coupled 808-nm LD, and the pigtail fiber had a core diameter of 400 μm and an NA of 0.22. The pump radiation was collimated by lens L1 and then was focused on the gain medium of the laser by lens L2 with a measured spot size of 760 μm. The focal lengths of L1 and L2 were 25.4 and 40 mm, respectively.
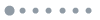
Figure 1.Experimental setup of maglev rotating Nd:YAG disk laser.
The gain medium used was a piece of an Nd:YAG crystal disk, which had 1.0 at.% doping concentration of ions with the dimensions of 0.5 mm in thickness and 16 mm in diameter. This crystal disk’s upper surface was coated for high transmission at 808 nm and a reflectivity of 98% at 1064 nm, and its downward surface was coated for high reflectivity at 1064 nm. The optical round trip between the crystal disk’s two surfaces acted as the cavity of the laser resonator, with its upper face providing the output coupling.
A photograph of the maglev system in this experiment is shown in Fig. 2. As shown, the maglev system included a stack of permanent magnets and a disk of PG. The stack of permanent magnets consisted of one NdFeB rod magnet and five hollow NdFeB cylindrical magnets. The rod magnet had a height of 50 mm and a diameter of 12.3 mm. Each hollow cylindrical magnet had a height of 10 mm, an inner diameter of 12.7 mm, and an outer diameter of 30 mm, and they were piled up in the height direction. The rod magnet was inserted into the latter magnets with their magnetic poles opposite to each other. The PG disk had a thickness of 0.9 mm and a diameter of 15 mm, and the laser crystal was concentrically glued on its upper surface. They had an overall weight of 808.3 mg, and the combination was levitated above the stacked permanent magnets at a spacing of . As this combination was heavy, the rotational kinetic energy in the PG disk induced by the unabsorbed pump power was too small to drive its rotation. In this case, two nozzles, placed along the edge of the PG disk with centrosymmetrical and opposite directions, were used to feed the compressed nitrogen gas tangentially. In this way, the levitated compound began to rotate at a frequency of .
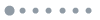
Figure 2.Photograph of the combination of the laser crystal and the PG levitated above a stack of permanent magnets.
The arrangement described above brought us a maglev rotating laser. In this laser, the pump spot on the rotating disk of the laser crystal was located at a centrifugal distance of about 6 mm. Further, the laser emission was directed by a dichroic mirror M2, which had a high transmission at 808 nm and high reflectivity at 1064 nm.
In the investigation, the combination of the laser crystal and the PG disk rotated at the frequency of . The laser started to oscillate when the absorbed pump power () exceeded 170 mW. It was also observed that the rotation speed of laser crystal and the PG became slower when the pump power increased.
The output power of this maglev rotating disk laser was detected by a power meter at a sampling rate of 0.3 s. Figure 3 depicts the variation of the measured laser power with the time at different pump powers. It can be seen that the real-time power fluctuated with the time around the corresponding mean value of the laser power, and the amplitude of the fluctuation increased with the pump power. As the size of the pump spot in the Nd:YAG crystal was bigger than the thickness of the crystal, the effect of disk wobbling on the laser performance was reduced significantly. Thus, the fluctuation in the laser power was mainly associated with the quality imperfection of the laser crystal and also the disturbance of thermal-induced stress in the thin Nd:YAG laser cavity.
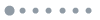
Figure 3.Time variation of the output power of the maglev rotating disk laser at different pump powers.
Figure 4 plots the measured power of the laser as a function of the absorbed pump power. As can be seen, with the increase of the pump power, the laser power increased linearly with a slope efficiency of 4.2% at a lower pump power () and 8.3% at a higher pump power (), and reached 17.7 mW at . The increase of the laser slope efficiency at a higher pump power can be attributed to the fact that the rotating speed at the higher pump power became close to the optimal rotation speed to achive the maximum output power from a rotary disk laser[8,14]. Further, the laser power could be improved by using a more flexible valve to precisely control the jet velocity of the compressed nitrogen gas to make the disk rotate at an optimal speed. In addition, the optimization of the reflectivity of the output coupler was another possible route to impove the power of this maglev rotating disk laser.
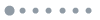
Figure 4.Laser power as a function of the absorbed pump power.
The beam profile was monitored by a CCD camera. Figure 5 shows the intensity distribution of the laser beam of the maglev rotating disk laser obtained at when the combination of the laser crystal and the PG was rotated at a frequency of . It can be seen that the intensity profiles in both the horizontal and vertical directions are near the Gaussian distribution with a measured beam diameter ( of peak intensity) of 1.3 mm; thus, the laser was operating at the near- mode. When the laser crystal and the PG were kept in the stationary state (without ejecting the compressed nitrogen gas), the intensity distribution of the laser beam was as shown in Fig. 6. It can be seen that the intensity distribution of the laser beam became diffusive with a measured beam diameter ( of peak intensity) of 2.4 mm, and that it did not keep in Gaussian shape in both the horizontal and vertical directions. The deterioration in the beam quality should be associated with the thermal accumulation in the laser crystal when the laser crystal and the PG were in the stationary state. Therefore, the results shown in Figs. 5 and 6 indicate that the laser beam obtained in the rotating state exhibits a higher brightness than that obtained in the stationary state.
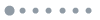
Figure 5.Intensity distribution of the laser beam at when the laser crystal and the PG were in the rotating state.
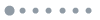
Figure 6.Intensity distribution of laser beam at when the laser crystal and the PG were in the stationary state.
In conclusion, we report a proof-of-concept investigation on an LD end-pumped maglev rotating Nd:YAG disk laser. In this laser, the laser crystal disk is attached to a PG disk. This combination is levitated above a permanent magnet stack and is driven to rotate by compressed nitrogen. With this maglev rotating laser, the detrimental thermal effects are alleviated, and thus the laser is operating in the single mode with high brightness. Future developmental work on this kind of laser may concentrate on scaling to a higher output power or realizing an optically driven maglev rotating disk laser. To obtain a higher output power, both the reflectivity of the output coupler and the rotating frequency should be optimized, and a big, thick crystal with a high doping concentration should be applied in the presence of a strong magnetic field. For an optically driven maglev rotating disk laser, the compound of the gain medium and the PG disk should become small, for example, like using the compound of a waveguide gain medium and a thin PG disk. It is expected the maglev rotating disk laser will facilitate many kinds of applications in the future.
References
[1] O. Puncken, H. Tünnermann, J. J. Morehead, P. Weßels, M. Frede, J. Neumann, D. Kracht. Opt. Express, 18, 20461(2010).
[2] A. K. Cousins. IEEE J. Quantum Electron., 28, 1057(1992).
[3] J. Frauchiger, P. Albers, H. P. Weber. IEEE J. Quantum Electron., 28, 1046(1992).
[4] S. Basu, R. L. Byer. App. Opt., 29, 1765(1990).
[5] S. Basu, R. L. Byer. Fiber coupled diode pumped moving solid state laser. U.S. Patent(1990).
[6] J. Korn, T. H. Jeys, T. Y. Fan. Opt. Lett., 16, 1741(1991).
[7] Y. Chen, V. Kushawaha. Appl. Phys. B, 61, 525(1995).
[8] S. Basu. IEEE J. Sel. Top. Quantum Electron., 11, 626(2005).
[9] S. Basu, M. Camargo, J. Donnelly. Proc. SPIE, 5707, 251(2005).
[10] S. Basu, B. Norton. Proc. SPIE, 6100, 61001M(2006).
[11] R. E. Pelrine. Am. Sci., 92, 428(2004).
[12] Q. Li, K. S. Kim, A. Rydberg. Rev. Sci. Instrum., 77, 065105(2006).
[13] M. Kobayashi, J. Abe. J. Am. Chem. Soc., 134, 20593(2012).
[14] S. Basu, A. Sridharan. Opt. Express, 12, 3114(2004).