
- Chinese Optics Letters
- Vol. 19, Issue 12, 122702 (2021)
Abstract
1. Introduction
The unique properties of qubits governed by quantum mechanics laws provide us with new methods for secure communication with unparalleled information processing capability[
The ultimate goal of quantum communication is to establish a global quantum Internet[
Although QKD research has made remarkable progress[
Sign up for Chinese Optics Letters TOC. Get the latest issue of Chinese Optics Letters delivered right to you!Sign up now
Photons are the natural physical carriers of quantum information since they are convenient to be prepared, manipulated, and transferred[
There is a consensus that the future global quantum network cannot be realized depending on a single scheme[
At present, most of the terrestrial free-space QKD experiments are performed in stationary line-of-sight links. Although satellites are good candidates for providing QKD service over 1000 km or even at the intercontinental scale, daunting costs and limited operation time windows of satellites hinder their massive deployment.
Due to the line-of-sight propagation feature of photonic signals, the free-space links cannot bypass physical obstructions such as mountains, buildings, and trees. Besides, these links may be blocked by interference like fog and clouds. Therefore, establishing the mobile nodes and links as demanded is essential to satisfy the requirement of practical QKD applications.
Airborne platforms or high-altitude platforms (HAPs) are ideal mobile nodes that can synergize with terrestrial links and quantum satellites to build a global quantum network. This review discusses QKD and entanglement distribution systems based on the aircraft in free-space channels. First, we introduce the basic concept and progress of airborne QKD experiments. Second, the salient advantages and critical techniques of airborne quantum communications are summarized. The third part highlights the future challenges and perspectives, and we conclude in the final part.
2. Concept and Progress
In this section, we focus on the basic concepts and recent progress of airborne QKD. We also discuss the advantages of adopting the airborne platform for quantum communications.
2.1. Basic concept
Airborne QKD refers to specific schematics to achieve quantum communication in free-space targeting short-range, local-area networks, and mobile scenarios. Compared with terrestrial free-space experiments, airborne QKD features dynamic optical links due to drastic relative motions between the transmitter and receiver. Figure 1 shows the blueprint of a hierarchical quantum network operating in different atmospheric layers. The satellite QKD aims at establishing quantum channels at the scale of thousands of kilometers. Generally, a lower altitude satellite benefits from lower diffraction-induced losses and launching cost. However, the line-of-sight timing window will be shorter, and the acquisition, tracking, and pointing (ATP) system should be more accurate and faster. Hence, the communication efficiency, duration, complexity, and cost should be balanced with synthetic considerations, and all available technologies should be optimally integrated to build the global quantum network.
Figure 1.Hierarchical quantum network operating in different atmospheric layers. LEO, low Earth orbit; MEO, medium Earth orbit; GEO, geostationary Earth orbit; HAP platform, high-altitude platform; UAV, unmanned aerial vehicle.
Similar to the satellite QKD, diverse flying vehicles have different characteristics and their optimum application scenarios. The physical height limit of a drone with spinning propellers is around 10 km. However, the off-the-shelf drones commonly fly below 500 m under normal conditions. The moving platforms like the manned/unmanned aircraft and helicopters fly in the region of 5–15 km, while the floating vehicles such as hot-air balloons and HAPs work above 15 km[
Airborne quantum nodes like drones or unmanned aerial vehicles (UAVs) could serve as temporary relays to solve the last-mile quantum key exchange for an inner-city or a field network benefiting from their rapid deployment capabilities. For higher altitude, the low-velocity aircraft is advantageous to provide longer link duration and broader transmission coverage. Quantum communication based on this platform can serve as a tactical approach to secure confidential data at a larger scale. Beyond the height of the troposphere, airborne platforms can assist in relaying the quantum signals from satellites to the ground or vice versa.
In general, airborne systems present a flexible approach for expanding the scope of quantum communications in time and space. Within the context of intercontinental satellite links, which have been verified[
2.2. Recent progress on airborne quantum communications
Several groups have reported their research results in airborne quantum communications in recent years, including the air-to-ground or backlink QKD between a rapidly moving aircraft and a ground station[
Figure 2.Recent progress in airborne quantum communications. In clockwise order, the first downlink QKD demonstration in 2013 using the hot-air balloon by Wang et al.[
In the view of recent experiments, airborne platforms are primarily used to simulate the altitude variations and relative motions of a satellite to evaluate the feasibility of spaceborne QKD sessions. However, with the rapid growth of the UAV industry and the development of automatic control technologies, UAVs or drones have become popular in the military, rescue, metrology, and even logistics and delivery[
What is more, Vasylyev et al. investigated the statistical features of quantum light to account for the influence of atmospheric turbulence and random scattering caused by raindrops[
Table 1 shows a summary of the recent progress of airborne QKD and related airborne projects. The following section will discuss the critical technologies implementing airborne QKD and discuss the issues that may need to be improved in the next step.
Initiative | Platform | Distance and Loss | Height | Velocity (km/h) | Results | Wavelength (nm) | System Clock Rate (MHz) | Quantum Signal Detector | Operation Time |
---|---|---|---|---|---|---|---|---|---|
Wang et al., 2013[ | Hot-air balloon | 20 km | N/A | 0 | Downlink, polarization-coded BB84 268.87 bps (secure key) | 850 | 100 | Si avalanche photodiode (APD) | Night |
Nauerth et al., 2013[ | Dornier-228 | 20 km | 1.1 km | 290 | Downlink, polarization-coded BB84 145 bps (sifted key) | 850 | 10 | Si APD | After sunset |
38 dB | |||||||||
Zhang et al., 2014[ | Z-9 | 2.5–7.5 km | 100 | Downlink (polarization basis detection and compensation) | 850 | 1 | Si APD | Night | |
Pugh et al., 2017[ | Twin Otter | 3–10 km | 1.6 km | 198–259 | Uplink, polarization-coded BB84 263.7–347 bps (secure key) | 785 | 400 | Si APD | Night |
34.4–51.1 dB | |||||||||
Liu et al., 2020[ | UAV | 200 m 12 dB | 0 | Downlink polarization entanglement distribution (CHSH-S parameter | 810 | N/A | Si APD | Daytime/clear/rainy night | |
Alexander et al., 2017[ | UAV (DJI S1000+) | N/A | N/A | Downlink, project: polarization-encoded BB84 | 650 | 100 | Si APD | Indoor/outdoor night | |
10–20 dB | |||||||||
Quintana et al., 2019[ | UAV | 1 km | N/A | N/A | Downlink/uplink, project: BB84 based on photonic integrated circuits (PICs) | 1550 | N/A | Indium gallium arsenide (InGaAs) detector in Geiger mode | N/A |
Liu et al., 2021[ | UAV | ∼1 km | 0 | Relayed entanglement distribution (CHSH-S parameter | 810 | N/A | Si APD | Night | |
20 dB |
Table 1. List of Recent Airborne Quantum Communication Experiments and Related Projects
2.3. Advantages of applications
Airborne platforms can provide a unique environment to explore the boundaries of quantum theory in aeronautical environments with microscopic particles or atmospheric factors, which are inaccessible in the laboratory[
The most important advantage of the airborne QKD lies in its high deployment flexibility and maneuverability. Aircrafts require no existing fiber channel and are not restricted by predictable trajectories and complicated launching activities compared with satellites. Consequently, the reusability and long endurance of aircrafts allow the QKD links to be deployed in remote areas on demand and have promise in extending the available working duration in cooperation with the satellite-based QKD.
The next advantage of airborne QKD is robustness against diverse weather conditions. In order to realize a wide-area all-weather quantum communication network, airborne platforms should avoid being blocked by clouds, haze, or terrible weather by traveling to the sites that are not affected by the weather. The mobility of the airborne platform can assist the system in searching a link with lower background noises. To this end, Liu et al. tested the S-parameter of the Clauser–Horne–Shimony–Holt (CHSH-S) inequality in their drone-based entanglement distribution experiment during clear daytime, clear night, and rainy night[
In general, there are two ways to help airborne QKD extend its communication distance and cope with more complex channel conditions. The first one is to utilize the relatively stable flying platforms, which have better loading capacities, and then more complex optical devices with larger apertures and adaptive optics can be adopted. Platforms like hot-air balloons or airplanes have shown the potential to carry out real-life QKD over the channel distance of about 20 km, as shown in Table 1. Secondly, it is possible to cover a wider area or tolerate more complex weather conditions by integrating numbers of airborne nodes into a network as relays or terminals. This scheme has been demonstrated by Liu et al. with the experiment of entanglement distribution[
3. System Design and Kernel Technologies
Free-space QKD is now experiencing a comprehensive evolution towards a reconfigurable and integrated heterogeneous network exploiting multiple types of channels. Despite the numerous theoretical and experimental achievements for satellite[
3.1. System composition
Similar to the terrestrial free-space QKD systems, a typical airborne QKD system can be divided into the following modules according to their functions: quantum source, detector, optical calibration with the ATP, classical communication, and post-processing. Compared with the ground-based free-space experiments, the most dramatic difference lies in the additional integration platforms and multi-stage ATP mechanisms. In ground-based QKD systems, some optical components or detection devices are deployed in detached positions to avoid background noise[
Figure 3 shows the block diagrams of an airborne QKD system. The red dotted blocks present the quantum source and detector on each side. They are the core components for qubits preparation and measurement, which usually comprise the laser source, intensity, polarization or phase modulators, quantum random number generators, single-photon detectors, and other auxiliary devices for system compensation.
Figure 3.Block diagrams of airborne QKD system. QRNG, quantum random number generator; Mod, modulator; Aux, auxiliary devices; TDC, time-to-digital converter; ATP, acquisition, tracking, and pointing; FSM, fast-steering mirror; PSD, position-sensitive detector; C, coupler; M, mirror; SPD, single-photon detector.
The imperfections of these devices may bring in side-channel loopholes and undermine the security of the practical QKD sessions. For the quantum source module, the parameters of the output signals like frequency, wavelength, line width, and intensity should be kept stable and consistent during the transmission procedure to avoid source-dependent attacks. For example, in an air-to-ground QKD experiment[
The blue dotted blocks represent the optical calibration and ATP modules, which stabilize the optical link and maintain maximal coupling efficiency between remote terminals. The ATP modules are usually made up of coarse and fine pointing feedback loops according to the requirements of the specific applications. The coarse pointing loop aims at initial alignment for the beacon laser within the receiving field-of-view (FOV) and features in the wide scanning range and low bandwidth. The fine pointing loop is used for the accurate alignment and residual error correction after coarse pointing. As shown in Fig. 3, a position-sensitive detector (PSD), fast-steering mirror (FSM), and motor are typical apparatus to constitute a course pointing loop. ATP systems can be classified into the gimbal-based, mirror-based, gimbal–mirror hybrid, and others, which have been well investigated in mobile FSO communications[
The gray blocks denote the classical communication and post-processing module, which have three major tasks. Firstly, this part will perform the basis reconciliation, error correction, and privacy amplification after the transmission of qubits is accomplished. Second, well-defined clock synchronization is also attributed to classical communication, which is beneficial to precise modulation and systematic coordination. Finally, all of the electronics used to support the QKD process and flying campaign are referred to as classical communications, including the time-to-digital converter, global positioning system (GPS) data transmission, command and control information distribution, etc.
The purple blocks indicate the hosting platforms that carry a transmitter or receiver. The way that they are connected to the QKD equipment is the major difference between the airborne QKD and other QKD systems. For the moment, there are three ways to install and integrate the optical antennas onto the airborne platforms. First, using an external hanging pod to protect the optical devices is the most straightforward and cost-effective way to carry the QKD assembly. However, the system stability can be easily influenced by vibrations during take-off and landing due to the rigid coupling. What is more, this paradigm will change the aerodynamic characteristics of the airborne platforms to some extent and lead to strong local turbulences, which poses great challenges on signal receiving and detection. The second way is to equip QKD devices inside the cabin of the platforms. In this manner, one can fix the transmitter or receiver on the floor of an aircraft or helicopter and mount the telescopes used for the beam direction facing outside with the cabin door open or removed. This approach is beneficial for real-time maintenance during flight and carries a heavy payload at the same time. Nonetheless, it has an obvious drawback in that the angular motion range of the telescope is limited due to the width of the cabin door[
3.2. Link configurations and the channel loss
Concerning different scenarios that allow the transmission of qubits, the airborne platforms can play the role of a transmitter, receiver, or even an optical relay node. Figure 4 illustrates three possible cases for airborne quantum communications. In the uplink configuration of Fig. 4(a), the quantum transmitter is either placed at the ground station or portable station, where line-of-sight links can be established with a moving aircraft[
Figure 4.Link configurations for airborne QKD.
In satellite QKD, the loss characteristics of the link and the receiver can be summarized as atmosphere attenuation, space coupling loss to the receiver telescope, the receiver’s telescope loss, and quantum receiver loss[
The atmospheric turbulence is an intractable barrier causing beam distortion and transmission efficiency fluctuation in free-space quantum channels, especially in the near-surface channel. Fortunately, the turbulence intensity characterized by the refractive index decreases with the smaller zenith angles[
Therefore, stratospheric platforms are slightly subjected to unstable airflow and turbulence. Moll et al. have analyzed the feasibility and preferability of implementing both discrete-variable QKD (DV-QKD) and continuous-variable QKD (CV-QKD) in the stratosphere[
Another important factor leading to the transmission loss of quantum communication in free space is the diffraction-induced beam broadening, which is determined by various factors such as the wavelength of the laser, the propagation distance, and the apertures of the transmitter and receiver. This is also known as geometric loss, a core parameter in evaluating the performance of QKD. In general, larger receiving apertures or smaller transmitting divergences result in less geometric loss for a given range according to the formula[
The asymmetric channel loss of different configurations with the same altitude can significantly differ in a satellite-based QKD system design and practical operation. Whereas airborne platforms fly at a much lower altitude than the LEO satellites, the total link budget has minor deviations between the uplink and downlink in terms of diffraction loss, atmospheric turbulence, and other types of attenuation. What should be noted is that one can achieve low diffraction loss within a long-distance path by dividing it into several short segments and exploiting the optical relay method[
3.3. Generating and manipulating high-quality quantum optical signals
Optical technologies are the most mature approaches to implement quantum communications, and the performance of the QKD system significantly depends on the quality of the light source. The ideal single-photon source that contains only one photon per pulse is the best security solution. Unfortunately, the ideal single-photon source is far from practically available, even if a few photons can be generated by quantum dots[
Polarization encoding is the preferred scheme to implement free-space QKD. For a polarization-encoded system, the polarization extinction ratio (PER) is the primary parameter to characterize the quality of the quantum signals and determines the intrinsic errors of the QKD system.
Figure 5 shows a comparison of the simulated key rate of a decoy-state polarization-encoded QKD system with different values of PER.
Figure 5.Secure key rates with different PER.
It is suggested that the PER of the transmitting signal in a free-space polarization-encoded QKD system should be higher than 20 dB[
In 2013, two representative airborne QKD experiments were reported simultaneously. The two groups from China and Germany both employed the four-laser diode quantum source scheme. The light source in Ref. [46] was composed of four laser diodes working at 850 nm. The two pairs of orthogonal states
In Ref. [44], they used four laser diodes, which are intrinsically linearly polarized (typically PER of 1000:1, i.e., 30 dB). Then, the emitted pulses encoded with four polarization states are driven by a 100 MHz clocking field programmable gate array (FPGA) that generates short pulses with 10 MHz repetition rate and 1 ns pulse width. It is intuitive and convenient to modulate multiple lasers separately or side-by-side. In this way, the SMF coupling method is indispensable for obtaining both spatial consistency and coaxiality. However, coupling to the SMF requires refined temperature stabilization and anti-vibration measures due to the polarization mode dispersion (PMD) effect. Wang et al. added a monitoring power meter to ensure that the pulse amplitude is consistent. In contrast, Nauerth et al. added narrow-band spatial filtering and a servo arm equipped with attenuator diodes to calibrate the pulse intensity in real-time.
It should be noted that if the multi-laser scheme is adopted, the parameters of photons from different lasers, such as the wavelength, repetition frequency, pulse width, PER, etc., should be kept indistinguishable to eliminate the risk of the side-channel loopholes[
In 2010, Jofre et al. proposed a free-space polarization-encoded QKD experiment using a single laser with a frequency of 100 MHz[
Using a single laser with multiple optoelectronic modulators is also an available solution for airborne QKD. From 2015 to 2017, the group led by Jennewein has conducted a series of QKD experiments based on mobile platforms, including truck and aircraft[
Figure 6.Quantum source and transmitter in the ground-to-air QKD demonstration[
An intensity modulator was used to modulate the photons to the signal states, decoy states, and vacuum states, respectively. A balanced Mach–Zehnder interferometer (MZI) with a phase modulator was used to modulate the photons to the horizontal, vertical, diagonal, and anti-diagonal polarization states.
3.4. High-precision ATP and polarization compensation
ATP is a kernel component to establish FSO links and maintain alignment between the transmitter and receiver. It has upgraded from two-dimensional gimbal-stage mechanisms to adaptive optics and liquid-crystal-based systems in the FSO system and has been reviewed comprehensively[
However, this is infeasible in quantum scenarios for security reasons, such as the no-cloning theorem of single photons. Reducing the background noise and keeping a steady high-efficiency optical alignment by using the ATP is a possible way to enhance the SNR. Secondly, polarization deflections are inevitable during long-distance free-space propagation with dramatic relative motions between two sides. Besides, the massive mirrors and coatings used in the ATP systems will introduce unnecessary birefringence to the quantum states[
For satellite-based QKD, the ATP system and its controlling algorithm can be designed according to the orbiting routine and flying data. For example, in the in-orbit test of the optical link of the satellite-to-ground QKD experiment[
The high-precision ATP system for quantum communication often adopts the gimbal mirror hybrid structure to perform the bidirectional tracking and pointing. Generally, two sets of control loops to perform the coarse and fine pointing sessions are contained on each end, and their tracking errors are typically about several hundred and several microradians, respectively.
The quantum beam’s divergence angle is commonly narrowed to enhance the link efficiency. The typical divergence value is approximately 180 µrad in the air-to-ground QKD experiment[
Representative Examples | Nauerth et al., 2013[ | Zhang et al., 2014[ | Liu et al., 2020[ | ||||
---|---|---|---|---|---|---|---|
Components | Transmitter | Receiver | Transmitter | Receiver | Transmitter | Receiver | |
Coarse pointing | Type | Torque | 2-axis | 2-axis | 2-axis | 3-axis | 3-axis |
motors | gimbal | gimbal | gimbal | gimbal | gimbal | ||
Tracking range | Azimuth | Azimuth | Azimuth | Azimuth | |||
Elevation | Elevation | Elevation | Elevation | ||||
Fine pointing | Type Range | VCM | PM | FSM | FSM | PZT FSM | PZT FSM |
Coarse camera | Type | InGaAs | InGaAs | CMOS | CMOS | CMOS | CMOS |
FOV | 48 mrad | 12.8 mrad | 2° ( | 1° ( | |||
Fine camera | Type | 4QD | InGaAs | CMOS | CMOS | PSD | PSD |
FOV | 3.3 mrad | 960 µrad | 512 µrad | 512 µrad | |||
Frame rate | 400 Hz | 2.3 kHz | 2.3 kHz | 60 kHz | 60 kHz | ||
Beacon laser | Divergence | 3 mrad | 1 mrad | 10 mrad | 10 mrad | ||
Tracking error | 500 µrad |
Table 2. ATP Performance of the Typical Airborne Quantum Communication Experiments
Before the ground-to-air QKD demonstration, Bourgoin et al.[
As for the drone-based entanglement distribution demonstration by Liu et al.[
Figure 7.ATP system in the drone-based entanglement distribution experiment[
The detection results are only significant to generate secure key bits when the transmitter and the receiver select the same bases. For example, in a polarization-encoded QKD system, the overall polarization degradation is mainly derived from the base deviations due to the relative motions of the transmitter and the receiver, which will make the so-called shared reference frames inconsistent. Unlike the stationary systems, in the mobile or airborne scenario, the high-fidelity transmission of the quantum states and the reference frame calibration are not trivial tasks to be accomplished or even critical technical challenges.
There are several schemes to improve the polarization fidelity of the quantum states of the transmitted photons. The first one is to optimize the design of the optical module either by minimizing the incident angle or making the mirrors and wave plate deposited in orthogonal positions to maximize the polarization visibility. This method was fully verified in the air-to-ground QKD demonstration[
The polarization compensation is an intuitive method adopted in airborne quantum communications. Pugh et al. designed a correcting system comprising a polarization tomography and motorized wave plate assembly at the transmitter[
The second manner was to carry out an open loop polarization compensation procedure based on carefully characterizing relevant system components, such as the static birefringence of the optics device in the aircraft and the ground station, the phase shifts introduced by the mirror with different light incident angle or the optical dome the light passed, and the ration around the beam axis introduced by the flight altitude of the aircraft. All of these decomposed distortions to the polarization can be either measured in advance or calculated from the live data of the flight terminal and the station. Therefore, the open loop method can be, in principle, performed without the auxiliary calibration light and additional intervals. Therefore, the open loop scheme is able to keep the quantum transmission uninterrupted, which is advantageous, especially in view of the finite-size effects. It is foreseeable that the practical effects of the open loop method will be related to the algorithm and the correctness of the pre-determined system parameters.
3.5. High-precision synchronization
Precise synchronization is indispensable for a QKD system for at least two reasons[
The synchronization methods such as electrical-signal synchronization[
As shown in Fig. 8, there is a fixed time offset
Figure 8.Schematic diagrams of time synchronization precision.
If the signal temporal expansion is too wide, the system cannot distinguish whether the incident light pulse is a dark count or a quantum signal within the coincidence window. Assume that the quantum bit error rate (QBER) can be attributed to imperfections, including the source error
4. Challenges and Perspectives
Free-space QKD is an attractive topic and is still in its beginning stage, which is far from wide-scale applications. However, it is foreseeable to be broadly applied soon, especially for military fields like secure communication, accurate navigation, and precise situational awareness[
Realizing real-time, all-weather, and global coverage QKD is the primary stage to build the quantum internet. In the next step, dividing long-range quantum links into many segments through quantum entangle distribution and quantum memory gradually comes into being. Airborne QKD will function as effective relaying nodes by getting rid of spatial and temporal constraints. In general, airborne quantum communication is not only a powerful measure to protect the existing wireless communication, but also an important means to accelerate the integration of quantum information technology with other technologies. At present, airborne quantum communication was only experimentally verified by a few groups. For fixed-wing aircrafts, the flying tests are mainly with the line or circular routines. For drone-based demonstration, the platform hovers in the air and did not perform complicated maneuvers or rapid movements. The technology readiness level (TRL) of airborne quantum communication is still in the low stages. It is necessary to improve several of the following aspects to make this technology more applicable in real life.
4.1. Precise, rapid, and adaptive ATP
Despite numerous researches of ATP in classical communications, the requirements of the small divergence angle, random relative motion, and high SNR in airborne quantum communication still pose technical challenges to improve its accuracy. As mentioned above, predetermined straight-line or arc-line trajectories or hovering is beneficial to reduce the initial capture time and continuous tracking of the mobile terminals. The gimbal-stage mirror or FSM controlled by the fast-executing proportion integration differentiation (PID) algorithms is qualified to reach high accuracy and low overhead.
However, in practical applications, the flight path is not always fixed. Natural objects may occasionally block the line-of-sight due to the flying path variations, which will interrupt the beam coupling. Consequently, ATP combined with adaptive optics is suggested to be used together with intelligent feedback algorithms to search the target automatically and establish the alignment accurately. Second, the varying wavefront distortion caused by the turbulent atmosphere should be suppressed when designing ATP. Moreover, the trade-off between the apertures of the pointing telescope and its angular resolution should be reflected according to the basic diffraction limitation[
Another critical technical challenge of airborne quantum communication is the random altitude change of the platform such as roll, pitch, and yaw, which increases the difficulty of optical signal receiving and detecting and puts forward higher requirements on ATP systems. According to the airborne QKD demonstrated experimentally by Pugh et al.[
4.2. Miniaturization of the airborne devices
The power and space consumptions may be trivial to terrestrial experiments but vital to the airborne platforms, especially for UAVs or drones with limited payload. It is usually quoted as the size, weight, and power (SWaP) constraints[
As mentioned above, increasing the transmitting and receiving apertures is an efficient way of reducing the diffraction loss in free-space QKD. However, large optical devices burden the airborne platforms with limited SWaP ability. Besides, silicon-based single-photon detectors and InGaAs-based detectors exhibit different performances in airborne quantum communications. The former has a wide responding spectrum that provides flexible wavelength choice for the signal laser, and the latter is suitable for infrared photons detection but has relatively low detecting efficiency. They are both preferable to the bulk superconducting detectors, considering the payload limit.
Generally, the performance of QKD relates to the SWaP capacity in practical scenarios. Therefore, miniaturization of the QKD systems based on small-sized or tiny components should be considered in the next step. Some groups have been devoted to developing handheld[
4.3. Mitigating the influence of aero-optical effects
In satellite-based QKD sessions, most of the beam propagation happens in outer space. However, the airborne platforms are constantly operating within the atmosphere where the influence of turbulence cannot be overlooked. The effects such as beam wander, beam broadening, and scintillation caused by turbulence are detrimental to the process of signal tracking and receiving. It is noteworthy that the interaction between the airflow and the fuselage or propeller is a significant turbulence source, with either fixed-wing aircraft or rotary-wing UAVs. In some extreme cases, this interaction can even form a boundary layer due to the viscosity effects of the air, which will cause drastic fluctuations of the local refractive index and coupling efficiency of the QKD systems[
Finally, in addition to technical revolutions, a mature airborne QKD system should develop the theoretical models and parameters optimizations to get the best performance by considering large statistical fluctuations due to the versatile characteristics of the airborne channel and the system itself.
5. Conclusion
The architecture of reconfigurable and long-distance hybrid QKD schemes represents a key strategy to the success of a full-fledged quantum network. At present, a number of research works on airborne quantum communications have been reported. The platforms have also been successfully exploited in some special applications, such as the verification of the satellite-based QKD.
The future airborne platforms will be enhanced with more sophisticated flying control techniques and finer optical wavefront reshaping methods. As the competitive edges of airborne quantum communication, the scalability and flexibility are destined to be upgraded from 100 m to nearly 20 km and from point-to-point links to complicated multi-node networks.
It is foreseeable that airborne quantum communications will play a fundamental role in the global secure quantum network, whether being exploited as an individual or transitional communication approach. Therefore, the research of airborne quantum communications remains a blue ocean, showing great opportunities to explore the boundaries of quantum mechanics.
References
[1] C. H. Bennett, D. P. DiVincenzo. Quantum information and computation. Nature, 404, 247(2000).
[2] A. Galindo, M. A. Martin-Delgado. Information and computation: classical and quantum aspects. Rev. Mod. Phys., 74, 347(2002).
[3] C. H. Bennett, G. Brassard. Quantum cryptography: public key distribution and coin tossing. Proceedings of IEEE International Conference on Computers, Systems, and Signal Processing, 175(1984).
[4] A. Ekert. Quantum cryptography based on Bell’s theorem. Phys. Rev. Lett., 67, 661(1991).
[5] V. Scarani, H. Pasquinucci, N. J. Cerf, M. Dušek, N. Lütkenhaus, M. Peev. The security of practical quantum key distribution. Rev. Mod. Phys., 81, 1301(2009).
[6] H.-K. Lo, M. Curty, K. Tamaki. Secure quantum key distribution. Nat. Photon., 8, 595(2014).
[7] C. E. Shannon. Communication theory of secrecy systems. Bell Syst. Tech. J., 28, 656(1949).
[8] B. Schneier, P. Sutherland. Applied Cryptography: Protocols, Algorithms, and Source Code in C(1995).
[9] P. W. Shor. Polynomial-time algorithms for prime factorization and discrete logarithms on a quantum computer. SIAM J. Comput., 26, 1484(1997).
[10] R. Raussendorf, H. J. Briegel. A one-way quantum computer. Phys. Rev. Lett., 86, 5188(2001).
[11] E. Diamanti, H.-K. Lo, B. Qi, Z.-L. Yuan. Practical challenges in quantum key distribution. NPJ Quantum Inf., 2, 16025(2016).
[12] H. J. Kimble. The quantum internet. Nature, 453, 1023(2008).
[13] S. Wehner, D. Elkouss, R. Hanson. Quantum internet: a vision for the road ahead. Science, 362, eaam9288(2018).
[14] J. Qiu. Quantum communications leap out of the lab. Nature, 508, 441(2014).
[15] L. Greenemeier. Election fix? Switzerland tests quantum cryptography(2007).
[16] F.-X. Xu, W. Chen, S. Wang, Z.-Q. Yin, Y. Zhang, Y. Liu. Field experiment on a robust hierarchical metropolitan quantum cryptography network. Chin. Sci. Bull., 54, 2991(2009).
[17] S. Wang, W. Chen, Z.-Q. Yin, Y. Zhang, T. Zhang, H.-W. Li. Field test of wavelength-saving quantum key distribution network. Opt. Lett., 35, 14(2010).
[18] S. Wang, W. Chen, Z.-Q. Yin, H.-W. Li, D.-Y. He, Y.-H. Li. Field and long-term demonstration of a wide area quantum key distribution network. Opt. Express, 22, 21739(2014).
[19] W. Chen, Z.-F. Han, T. Zhang, W. Hao, Z.-Q. Yin, F.-X. Xu. Field experiment on a “star type” metropolitan quantum key distribution network. IEEE Photon. Technol. Lett., 21, 575(2009).
[20] S.-K. Liao, W.-Q. Cai, J. Handsteiner, B. Liu, J. Yin, L. Zhang. Satellite-relayed intercontinental quantum network. Phys. Rev. Lett., 120, 030501(2018).
[21] S. Pirandola, U. L. Andersen, L. Banchi, M. Berta, D. Bunandar, R. Colbeck. Advances in quantum cryptography. Adv. Opt. Photon., 12, 1012(2020).
[22] S. Slussarenko, G. J. Pryde. Photonic quantum information processing: a concise review. Appl. Phys. Rev., 6, 041303(2019).
[23] W.-Y. Hwang. Quantum key distribution with high loss toward global secure communication. Phys. Rev. Lett., 91, 057901(2003).
[24] X.-B. Wang. Beating the photon-number-splitting attack in practical quantum cryptography. Phys. Rev. Lett., 94, 230503(2005).
[25] H.-K. Lo, X.-F. Ma, K. Chen. Decoy state quantum key distribution. Phys. Rev. Lett., 94, 230504(2005).
[26] S. L. Braunstein, S. Pirandola. Side-channel-free quantum key distribution. Phys. Rev. Lett., 108, 130502(2012).
[27] H.-K. Lo, M. Curty, B. Qi. Measurement-device-independent quantum key distribution. Phys. Rev. Lett., 108, 130503(2012).
[28] T. Sasaki, Y. Yamamoto, M. Koashi. Practical quantum key distribution protocol without monitoring signal disturbance. Nature, 509, 475(2014).
[29] S. Pirandola, R. Laurenza, C. Ottaviani, L. Banchi. Fundamental limits of repeaterless quantum commuications. Nat. Commn., 8, 15043(2017).
[30] M. Lucamarini, Z.-L. Yuan, J. Dynes, A. J. Shields. Overcoming the rate-distance limit of quantum key distribution without quantum repeaters. Nature, 557, 400(2018).
[31] A. Boaron, G. Boso, D. Rusca, C. Vulliez, C. Autebert, M. Caloz, M. Perrenoud, G. Gras, F. Bussières, M.-J. Li, D. Nolan, A. Martin, H. Zbinden. Secure quantum key distribution over 421 km of optical fiber. Phys. Rev. Lett., 121, 190502(2018).
[32] J.-P. Chen, C. Zhang, Y. Liu, C. Jiang, W.-J. Zhang, X.-L. Hu, J.-Y. Guan, Z.-W. Yu, H. Xu, J. Lin, M.-J. Li, H. Chen, H. Li, L. You, Z. Wang, X.-B. Wang, Q. Zhang, J.-W. Pan. Sending-or-not-sending with independent lasers: secure twin-field quantum key distribution over 509 km. Phys. Rev. Lett., 124, 070501(2020).
[33] C. Simon. Towards a global quantum network. Nat. Photon., 11, 678(2017).
[34] S. Pirandola, S. L. Braunstein. Unite to build a quantum Internet. Nature, 532, 169(2016).
[35] C. H. Bennett, F. Bessette, G. Brassard, L. Salvail, J. Smolin. Experimental quantum cryptography. J. Cryptol., 5, 3(1992).
[36] W. T. Buttler, R. J. Hughes, P. G. Kwiat, S. K. Lamoreaux, G. G. Luther, G. L. Morgan, J. E. Nordholt, C. G. Peterson, C. M. Simmons. Practical free-space quantum key distribution over 1 km. Phys. Rev. Lett., 81, 3283(1998).
[37] R. J. Hughes, J. E. Nordholt, D. Derkacs, C. G. Peterson. Practical free-space quantum key distribution over 10 km in daylight and at night. New J. Phys., 4, 43(2002).
[38] C. Kurtsiefer, P. Zarda, M. Halder, H. Weinfurter, P. M. Gorman, P. R. Tapster, J. G. Rarity. Quantum cryptography: a step towards global key distribution. Nature, 419, 450(2002).
[39] T. Schmitt-Manderbach, H. Weier, M. Fürst, R. Ursin, F. Tiefenbacher, T. Scheidl, J. Perdigues, Z. Sodnik, C. Kurtsiefer, J. G. Rarity, A. Zeilinger, H. Weinfurter. Experimental demonstration of free-space decoy-state quantum key distribution over 144 km. Phys. Rev. Lett., 98, 010504(2007).
[40] J. Yin, Y. Cao, Y.-H. Li, S.-K. Liao, L. Zhang, J.-G. Ren, W.-Q. Cai, W.-Y. Liu, B. Li, H. Dai, G.-B. Li, Q.-M. Lu, Y.-H. Gong, Y. Xu, S.-L. Li, F.-Z. Li, Y.-Y. Yin, Z.-Q. Jiang, M. Li, J.-J. Jia, G. Ren, D. He, Y.-L. Zhou, X.-X. Zhang, N. Wang, X. Chang, Z.-C. Zhu, N.-L. Liu, Y.-A. Chen, C.-Y. Lu, R. Shu, C.-Z. Peng, J.-Y. Wang, J.-W. Pan. Satellite-based entanglement distribution over 1200 kilometers. Science, 356, 1140(2017).
[41] S.-K. Liao, W.-Q. Cai, W.-Y. Liu, L. Zhang, Y. Li, J.-G. Ren, J. Yin, Q. Shen, Y. Cao, Z.-P. Li, F.-Z. Li, X.-W. Chen, L.-H. Sun, J.-J. Jia, J.-C. Wu, X.-J. Jiang, J.-F. Wang, Y.-M. Huang, Q. Wang, Y.-L. Zhou, L. Deng, T. Xi, L. Ma, T. Hu, Q. Zhang, Y.-A. Chen, N.-L. Liu, X.-B. Wang, Z.-C. Zhu, C.-Y. Lu, R. Shu, C.-Z. Peng, J.-Y. Wang, J.-W. Pan. Satellite-to-ground quantum key distribution. Nature, 549, 43(2017).
[42] Y.-A. Chen, Q. Zhang, T.-Y. Chen, W.-Q. Cai, S.-K. Liao, J. Zhang, K. Chen, J. Yin, J.-G. Ren, Z. Chen, S.-L. Han, Q. Yu, K. Liang, F. Zhou, X. Yuan, M.-S. Zhao, T.-Y. Wang, X. Jiang, L. Zhang, W.-Y. Liu, Y. Li, Q. Shen, Y. Cao, C.-Y. Lu, R. Shu, J.-Y. Wang, L. Li, N.-L. Liu, F. Xu, X.-B. Wang, C.-Z. Peng, J.-W. Pan. An integrated space-to-ground quantum communication network over 4600 kilometers. Nature, 589, 214(2021).
[43] F. Fidler, M. Knapek, J. Horwath, W. R. Leeb. Optical communications for high-altitude platforms. IEEE J. Sel. Top. Quantum Electron., 16, 1058(2010).
[44] S. Nauerth, F. Moll, M. Rau, C. Fuchs, J. Horwath, S. Frick, H. Weinfurter. Air-to-ground quantum communication. Nat. Photon., 7, 382(2013).
[45] C. J. Pugh, S. Kaiser, J. P. Bourgoin, J. Jin, N. Sultana, S. Agne, E. Anisimova, V. Makarov, E. Choi, B. L. Higgins. Airborne demonstration of a quantum key distribution receiver payload. Quantum Sci. Technol., 2, 024009(2017).
[46] J.-Y. Wang, B. Yang, S.-K. Liao, L. Zhang, Q. Shen, X.-F. Hu, J.-C. Wu, S.-J. Yang, H. Jiang, Y.-L. Tang, B. Zhong, H. Liang, W.-Y. Liu, Y.-H. Hu, Y.-M. Huang, B. Qi, J.-G. Ren, G.-S. Pan, J. Yin, J.-J. Jia, Y.-A. Chen, K. Chen, C.-Z. Peng, J.-W. Pan. Direct and full-scale experimental verifications towards ground–satellite quantum key distribution. Nat. Photon., 7, 387(2013).
[47] Y. Chu, R. Donaldson, R. Kumar, D. Grace. Feasibility of quantum key distribution from high altitude platforms(2020).
[48] H.-Y. Liu, X.-H. Tian, C.-S. Gu, P.-F. Fan, X. Ni, R. Yang, J.-N. Zhang, M.-Z. Hu, J. Guo, X. Cao, X. Hu, G. Zhao, Y.-Q. Lu, Y.-X. Gong, Z. Xie, S.-N. Zhu. Drone based quantum entanglement distribution towards mobile quantum network. Natl. Sci. Rev., 7, 921(2020).
[49] H.-Y. Liu, X.-H. Tian, C.-S. Gu, P.-F. Fan, X. Ni, R. Yang, J.-N. Zhang, M.-Z. Hu, J. Guo, X. Cao, X. Hu, G. Zhao, Y.-Q. Lu, Y.-X. Gong, Z. Xie, S.-N. Zhu. Optical-relayed entanglement distribution using drones as mobile nodes. Phys. Rev. Lett., 126, 020503(2021).
[50] M. Zhang, L. Zhang, J.-C. Wu, S.-J. Yang, X. Wan, Z.-P. He, J.-J. Jia, D. S. Citrin, J.-Y. Wang. Detection and compensation of basis deviation in satellite-to-ground quantum communications. Opt. Express, 22, 9871(2014).
[51] A. D. Hill, J. Chapman, K. Herndon, C. Chopp, D. J. Gauthier, P. Kwiat. Drone-based quantum key distribution. Urbana, 51, 61801(2017).
[52] A. Conrad, S. Isaac, R. Cochran, D. Sanchez-Rosales, B. Wilens, A. Gutha, T. Rezaei, D. J. Gauthier, P. Kwiat. Drone-based quantum key distribution: QKD. Proc. SPIE, 11678, 116780X(2021).
[53] A. D. Hill. Spatial mode control and advanced methods for multi-platform quantum communication(2018).
[54] J. P. Bourgoin, B. L. Higgins, N. Gigov, C. Holloway, C. J. Pugh, S. Kaiser, M. Cranmer, T. Jennewein. Free-space quantum key distribution to a moving receiver. Opt. Express, 23, 33437(2015).
[55] H. Shakhatreh, A. H. Sawalmeh, A. Al-Fuqaha, Z.-C. Dou, E. Almaita, I. Khalil. Unmanned aerial vehicles (UAVs): a survey on civil applications and key research challenges. IEEE Access, 7, 48572(2019).
[56] B. Li, Z.-S. Fei, Y. Zhang, M. Guizani. Secure UAV communication networks over 5G. IEEE Wireless Commun., 26, 114(2019).
[57] B. Qi, H.-K. Lo, C. C. W. Lim, G. Siopsis, E. A. Chitambar, R. Pooser, P. G. Evans, W. Grics. Free-space reconfigurable quantum key distribution network. 2015 IEEE International Conference on Space Optical Systems and Applications (ICSOS), 1(2015).
[58] D. Vasylyev, A. A. Semenov, W. Vogel. Free-space quantum links under diverse weather conditions. Phys. Rev. A, 96, 043856(2017).
[59] C. Quintana, P. Sibson, G. Erry, Y. Thueux, E. Kingston, T. Ismail, G. Faulkner. Low size, weight and power quantum key distribution system for small form unmanned aerial vehicles. Proc. SPIE, 10910, 1091014(2019).
[60] C. Agnesi, F. Vedovato, M. Schiavon, D. Dequal, L. Calderaro, M. Tomasin, D. G. Marangon, A. Stanco, V. Luceri, G. Bianco, G. Vallone, P. Villoresi. Exploring the boundaries of quantum mechanics: advances in satellite quantum communications. Trans. Royal Soc. A, 376, 20170461(2018).
[61] D. Rideout, T. Jennewein, G. A. Camelia, T. F. Demarie, B. L. Higgins, A. Kempf. Fundamental quantum optics experiments conceivable with satellites—reaching relativistic distances and velocities, class. Quantum Grav., 29, 224011(2012).
[62] R. Ursin, T. Jennewein, J. Kofler, J. M. Perdigues, L. Cacciapuoti, C. J. de Matos, M. Aspelmeyer. Space-quest, experiments with quantum entanglement in space. Europhys. News, 40, 26(2009).
[63] S. K. Joshi, J. Pienaar, T. C. Ralp, L. Cacciapuoti, W. McCutcheon, J. Rarity, D. Giggenbach, J. G. Lim. Space QUEST mission proposal: experimentally testing decoherence due to gravity. New J. Phys., 20, 063016(2018).
[64] R. Bedington, J. M. Arrazola, A. Ling. Progress in satellite quantum key distribution. NPJ Quantum Inf., 3, 30(2017).
[65] M. J. García-Martínez, N. Denisenko, D. Soto, D. Arroyo, A. B. Orue, V. Fernandez. High-speed free-space quantum key distribution system for urban daylight applications. Appl. Opt., 52, 3311(2013).
[66] Y. Kaymak, R. Rojas-Cessa, J. Feng, N. Ansari, M.-C. Zhou, T.-R. Zhang. A survey on acquisition, tracking, and pointing mechanisms for mobile free-space optical communications. IEEE Commun. Surv. Tutor., 20, 1104(2018).
[67] Y. Saito, H. Takenaka, K. Shiratama, Y. Munemasa, A. Carrasco-Casado, P. V. Trinh, K. Suzuki, T. Fuse, Y. Takahashi, T. Kubo-oka, M. Toyoshima. Research and development of a transportable optical ground station in NICT: the results of the first performance test. Front Phys-Lausanne, 9, 84(2021).
[68] F. Marsili, V. B. Verma, J. A. Stern, S. Harrington, A. E. Lita, T. Gerrits, I. Vayshenker, B. Baek, M. D. Shaw, R. P. Mirin, S. W. Nam. Detecting single infrared photons with 93% system efficiency. Nat. Photon., 7, 210(2013).
[69] P. Hu, H. Li, L.-X. You, H.-Q. Wang, Y. Xiao, J. Huang, X.-Y. Yang, W.-J. Zhang, Z. Wang, X.-M. Xie. Detecting single infrared photons toward optimal system detection efficiency. Opt. Express, 28, 36884(2020).
[70] H. Takenaka, A. Carrasco-Casado, M. Fujiwara, M. Kitamura, M. Sasaki, M. Toyoshima. Satellite-to-ground quantum-limited communication using a 50-kg-class microsatellite. Nature Photon., 11, 502(2017).
[71] D. Vasylyev, W. Vogel. Satellite-mediated quantum atmosphere links. Phy. Rev. A, 99, 053830(2019).
[72] J. P. Bourgoin, E. Meyer-Scott, B. L. Higgin, B. Helou, C. Erven, H. Hübel, B. Kumar, D. Hudson, I. D’Souza, R. Girard, R. Laflamme, T. Jennewein. A comprehensive design and performance analysis of low Earth orbit satellite quantum communication. New J. Phys., 15, 023006(2013).
[73] F. Moll, T. Botter, C. Marquardt, D. Pusey, A. Shrestha, A. Reeves, K. Jaksch, K. Gunthner, O. Bayraktar, C. Mueller-Hirschkorn. Stratospheric QKD: feasibility analysis and free-space optics system concept. Proc. SPIE, 11167, 111670H(2019).
[74] S. Bloom, E. Korevaar, J. Schuster, H. Willebrand. Understanding the performance of free-space optics. J. Opt. Netw., 2, 178(2003).
[75] M. Aspelmeyer, T. Jennewein, A. Zeilinger. Long-distance quantum communication with entangled photons using satellites. IEEE J. Sel. Top. Quantum Electron., 9, 1541(2003).
[76] M. Sasaki. Quantum networks: where should we be heading. Quantum Sci. Technol., 2, 020501(2017).
[77] R. Ramanathan, J. Red. A brief overview of ad hoc networks: challenges and directions. IEEE Commun Mag., 40, 20(2002).
[78] P. Senellart, G. Solomon, A. White. High-performance semiconductor quantum-dot single-photon sources. Nat. Nanotechnol., 12, 1026(2017).
[79] L. A. Ngah, O. Alibart, L. Labonté, V. D’Auria, S. Tanzilli. Ultra-fast heralded single photon source based on telecom technology. Laser Photon. Rev., 9, L1(2015).
[80] Y. Zhao, B. Qi, H.-K. Lo. Experimental quantum key distribution with active phase randomization. Appl. Phys. Lett., 90, 044106(2007).
[81] S.-H. Sun, L.-M. Liang. Experimental demonstration of an active phase randomization and monitor module for quantum key distribution. Appl. Phys. Lett., 101, 071107(2012).
[82] X. Han, H.-L. Yong, P. Xu, K.-X. Yang, S.-L. Li, W.-Y. Wang, H.-J. Xue, F.-Z. Li, J.-G. Ren, C.-Z. Peng, J.-W. Pan. Polarization design for ground-to-satellite quantum entanglement distribution. Opt. Express, 28, 369(2020).
[83] C. Z. Peng, J. Zhang, D. Yang, W.-B. Gao, H.-X. Ma, H. Yin, H.-P. Zeng, T. Yang, X.-B. Wang, J.-W. Pan. Experimental long-distance decoy-state quantum key distribution based on polarization encoding. Phys. Rev. Lett., 98, 010505(2007).
[84] S. Nauerth, M. Furst, T. Schmitt-Manderbach, W. Henning, W. Harald. Information leakage via side channels in freespace BB84 quantum cryptography. New J. Phys., 11, 065001(2009).
[85] H. Ko, B.-S. Choi, J.-S. Choe, K.-J. Lim, J.-H. Kim, C. J. Youn. High-speed and high-performance polarization-based quantum key distribution system without side channel effects caused by multiple lasers. Photon. Res., 6, 214(2018).
[86] M. Jofre, A. Gardelein, G. Anzolin, G. Molina-Terriza, J. P. Torres, M. W. Mitchell, V. Pruneri. 100 MHz amplitude and polarization modulated optical source for free-space quantum key distribution at 850 nm. J. Lightwave Technol., 28, 2572(2010).
[87] Z. Yan, E. Meyer-Scott, J.-P. Bourgoin, B. L. Higgins, N. Gigov, A. MacDonald, H. Hübel, T. Jennewein. Novel high-speed polarization source for decoy-state BB84 quantum key distribution over free space and satellite links. J. Lightwave Technol., 31, 1399(2013).
[88] C. Bonato, M. Aspelmeyer, T. Jennewein, C. Pernechele, P. Villoresi, A. Zeilinger. Influence of satellite motion on polarization qubits in a Space-Earth quantum communication link. Opt. Express, 14, 10050(2006).
[89] C. Bonato, C. Pernechele, P. Villoresi. Influence of all-reflective optical systems in the transmission of polarization-encoded qubits. J. Opt. A, 9, 899(2007).
[90] L. Zhang, J.-J. Jia, S.-K. Liao, G.-H. Wen, R. Shu, J.-W. Pan. Establishment and in-orbit test of optical link in satellite-to-ground quantum communication. Sci. China: Inf. Science, 48, 1183(2018).
[91] A. Laing, V. Scarani, J. G. Rarity, J.-L. O’Brien. Reference-frame-independent quantum key distribution. Phys. Rev. A, 82, 012304(2010).
[92] Y. Xue, L. Shi, W. Chen, Z.-Q. Yin, G.-J. Fan, H.-Y. Fu, Q.-H. Lu, J.-H. Wei. Improving the performance of reference-frame-independent quantum key distribution through a turbulent atmosphere. Phys. Rev. A, 102, 062602(2020).
[93] C. Agnesi, M. Avesani, L. Calderaro, A. Stanco, G. Foletto, M. Zahidy, A. Scriminich, F. Vedovato, G. Vallone, P. Villoresi. Simple quantum key distribution with qubit-based synchronization and a self-compensating polarization encoder. Optica, 7, 284(2020).
[94] T. Ferreir, D. Vitoreti, G. B. Xavier, G. C. do Amaral, G. P. Temporão, J. P. von der Weid. Proof-of-principle demonstration of measurement-device-independent quantum key distribution using polarization qubits. Phys. Rev. A, 88, 052303(2013).
[95] J. C. Bienfang, A. J. Gross, A. Mink, B. J. Hershman, A. Nakassis, X. Tang, R. Lu, D. H. Su, C. W. Clark, C. J. Williams. Quantum key distribution with 1.25 Gbps clock synchronization. Opt. Express, 12, 2011(2004).
[96] I. Marcikic, A. Lamas-Linares, C. Kurtsiefer. Free-space quantum key distribution with entangled photons. Appl. Phys. Lett., 89, 101122(2006).
[97] C.-Z. Peng, T. Yang, X.-H. Bao, J. Zhang, X.-M. Jin, F.-Y. Feng, B. Yang, J. Yang, J. Yin, Q. Zhang, N. Li, B.-L. Tian, J.-W. Pan. Experimental free-space distribution of entangled photon pairs over 13 km: towards satellite-based global quantum communication. Phys. Rev. Lett., 94, 150501(2005).
[98] R. Quan, Y.-W. Zhai, M.-M. Wang, F.-Y. Hou, S.-F. Wang, X. Xiang, T. Liu, S.-G. Zhang, R.-F. Dong. Demonstration of quantum synchronization based on second-order quantum coherence of entangled photons. Sci. Rep., 6, 30453(2016).
[99] A. Pljonkin, K. Rumyantsev, P. K. Singh. Synchronization in quantum key distribution(2018).
[100] J.-G. Ren, J. Yin, B. Yang, F. Zhou, Z.-H. Yi, C.-Z. Peng, R. Shu, J.-Y. Wang. Time synchronization for quantum key distribution form ground to satellite. J. Infrared Millim. Terahertz Waves, 30, 381(2011).
[101] N. M. P. Neumann, M. P. P. Heesch, P. Graaf. Quantum communication for military applications(2020).
[102] M. Krelina. Quantum warfare: definitions, overview and challenges(2021).
[103] Y. Zeng, R. Zhang, T. J. Lim. Wireless communications with unmanned aerial vehicles: opportunities and challenges. IEEE Commun. Mag., 54, 36(2016).
[104] S.-K. Liao, J. Lin, J.-G. Ren, W.-Y. Liu, J. Qiang, J. Yin, Y. Li, Q. Shen, L. Zhang, X.-F. Liang, H.-L. Yong, F.-Z. Li, Y.-Y. Yin, Y. Cao, W.-Q. Cai, W.-Z. Zhang, J.-J. Jia, J.-C. Wu, X.-W. Chen, S.-C. Zhang, X.-J. Jiang, J.-F. Wang, Y.-M. Huang, Q. Wang, L. Ma, L. Li, G.-S. Pan, Q. Zhang, Y.-A. Chen, C.-Y. Lu, N.-L. Liu, X. Ma, R. Shu, C.-Z. Peng, J.-Y. Wang, J.-W. Pan. Space-to-ground quantum key distribution using a small-sized payload on Tiangong-2 space lab. Chin. Phys. Lett., 34, 090302(2017).
[105] H. Chun, I. Choi, G. Faulkner, L. Clarke, B. Barber, G. George, C. Capon, A. Niskanen, J. Wabnig, D. O’Brien, D. Bitauld. Handheld free space quantum key distribution with dynamic motion compensation. Opt. Express, 25, 6784(2017).
[106] F.-X. Wang, W. Wang, R. Niu, X.-Y. Wang, C.-L. Zou, C.-H. Dong, B. E. Little, S. T. Chu, H. Liu, P.-L. Hao, S.-F. Liu, S. Wang. Quantum key distribution with on-chip dissipative Kerr soliton. Laser Photon. Rev., 14, 1900190(2020).
[107] P. Sibson, C. Erven, M. Godfrey, S. Miki, T. Yamashita, M. Fujiwara, M. Sasaki. Chip-based quantum key distribution. Nat. Commun., 8, 13984(2017).
[108] X. Zhang, B. A. Bell, A. Mahendra, C.-L. Xiong, P. H. W. Leong, B. J. Eggleton. Integrated silicon nitride time-bin entanglement circuits. Opt. Lett., 43, 3469(2018).
[109] Q. Gao, S.-H. Yi, Z.-F. Jiang, L. He, X.-H. Wang. Structure of the refractive index distribution of the supersonic turbulent boundary layer. Opt. Laser. Eng., 51, 1113(2013).
[110] J. P. Reid, A. K. Bertram, D. O. Topping, A. Laskin, S. T. Martin, M. D. Petters, F. D. Pope, G. Rovelli. The viscosity of atmospherically relevant organic particles. Nat. Commun., 9, 956(2018).
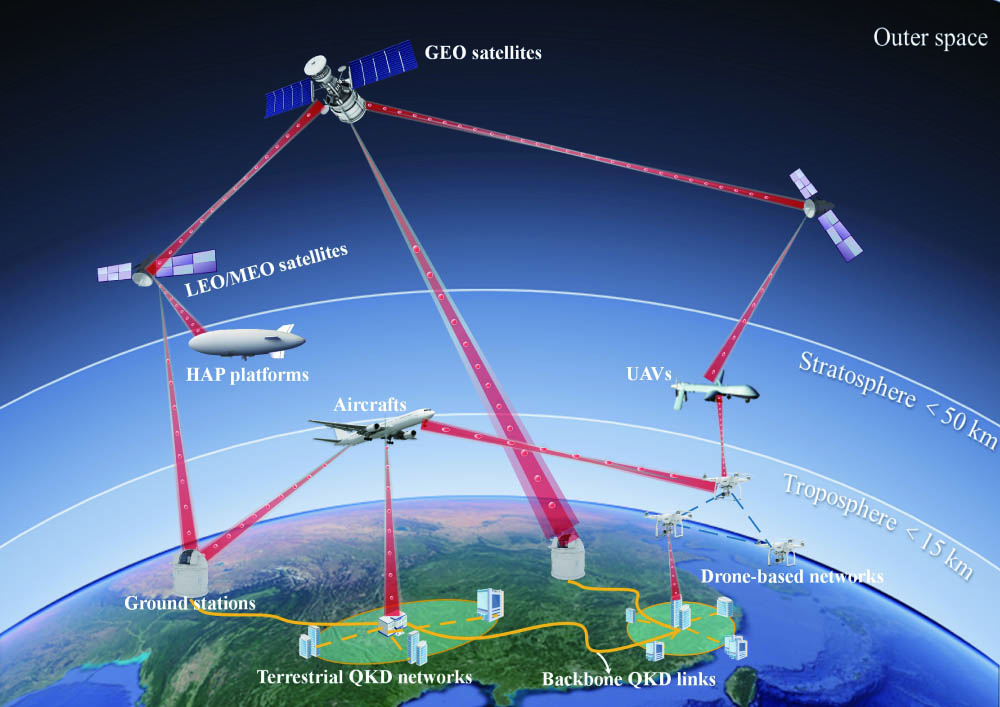
Set citation alerts for the article
Please enter your email address