
- Chinese Optics Letters
- Vol. 19, Issue 12, 120601 (2021)
Abstract
1. Introduction
The negatively charged nitrogen-vacancy (NV) centers in nanodiamonds (NDs) are a promising quantum sensor for various physical parameters such as magnetic field[
In this Letter, a facile integration method for an ND with a large number of NV centers has been developed. Specifically, NDs were mixed with UV adhesive in a proper ratio and then coated onto the fiber end surface. The UV adhesive had a very high adhesion to quartz material after curing[
2. Fabrication of Probe
The available NDs are produced by the high pressure and high temperature (HPHT) method, and the negatively charged NV centers are formed upon annealing of the irradiated nanocrystallites at 800°C for 2 h. On average, the N concentration is on the order of 100 ppm (parts per million), and the number of NVs is
Sign up for Chinese Optics Letters TOC. Get the latest issue of Chinese Optics Letters delivered right to you!Sign up now
As shown in Fig. 1(a), the manufacturing process of the probe is mainly divided into three steps. Step 1 is to dip the ND mixed UV adhesive on the end face of MMF2 (medium of optical fiber). Step 2 is to aim MMF1 (probe) at MMF2 and make the two end faces contact slowly. In step 3, MMF2 is moved in the opposite direction, and part of the UV adhesive remains on the end face of MMF1 due to adsorption, by controlling the contact area between the UV adhesive and the MMF1 end face, where hemispherical films of different thicknesses can be obtained.
Figure 1.(a) Fabrication steps of the sensing probe; (b) microscopic images of the sensor probes with different thickness film.
All of the above operations were carried out on a 3D displacement platform under a microscope. The film was finally cured after half an hour with a UV lamp, and the NDs were firmly fixed in the optical fiber end surface. Figure 1(b) shows three sensing probes with thickness (d) of (I) 9.20 µm, (II) 12.65 µm, and (III) 17.52 µm, respectively. The volume ratio of NDs in the film is only 1:175, and the NDs only account for a relatively small part. The number of NDs in the film can be easily tuned by changing the ratio of the NDs in the UV adhesive.
Figure 2 schematically depicts the experimental setup for optical measurement. A 532 nm laser was focused on the coated NDs on the end face of the MMF through a dichromatic mirror and lens, and the generated FL signal was then directed to the spectrometer through the original channel. A long pass filter (600 nm) was placed in front of the spectrometer (exposure time: 0.1 s) to filter out the pump laser.
Figure 2.Schematic diagram of the experimental setup.
3. Results and Discussion
In order to enhance the excitation and collection efficiency of FL signals, we coated an aluminum film with a thickness of 30–50 nm on the surface of the NDs film using the magnetron sputtering method. Figures 3(a)–3(c) show the microscopic images of the cross-section view of the uncoated fiber facet with only UV adhesive and aluminum film on the UV adhesive, respectively. As shown in Fig. 3(a), we can clearly see that the light reflected on the optical fiber facet is very uniform and bright when no material is coated, and, in Fig. 3(b), we can see the rough texture of the surface after curing of the UV adhesive. The spot in the center of the film was irregular because the reflected light was scattered by the rough surface. The brighter light spot in the center, as shown in Fig. 3(c), indicated the effective coating of the aluminum film as a reflective layer, which could also be seen by the SEM image shown in Fig. 3(d).
Figure 3.Microscopic images of the cross-section view of (a) uncoated fiber facet, (b) facet only coated with UV adhesive, and (c) facet with aluminum film coated on UV adhesive; (d) SEM image of the probe after aluminum film coating.
We compared the FL energy of the probe before and after coating in water. As shown in Fig. 4 under different excitation laser power, the FL energy collected by the aluminum film increased by 12.0%, 16.3%, and 22.4%, respectively. The FL signal enhancement might be caused by the following two reasons. First, the green light was reflected by the aluminum film, improving the excitation efficiency. Second, part of the excited FL is reflected by the aluminum film and then re-coupled into the optical fiber, further improving the collection efficiency. We believe that the collection efficiency can be further enhanced by optimizing the coating quality.
Figure 4.FL energy of probe I before and after coating with aluminum under the different excitation laser powers.
The impact of film thickness on the FL collection efficiency was investigated by simulation and experiment. The simulated results of FL collection using geometrical optics method are shown in Fig. 5. In the numerical investigation, we considered that the FL signal excited by NDs containing an NV center would be reflected back to the optical fiber after interacting with the aluminum film. The refractive indices of the diamond, optical fiber core, and cladding are set to be 2.4, 1.45, and 1.44, respectively. For the simulated FL collection shown in Fig. 5, we assumed five NDs evenly distributed in the UV adhesive. By changing the thickness of the film, it can be seen that the FL signal collected by the optical fiber core increases with the decrease of the radius of the hemispherical NDs coating. With the decrease of the radius of curvature, the convergence effect of aluminum film becomes more and more obvious, and therefore more FL signals are reflected back into the optical fiber core. Besides the curvature of the NDs coating, the number of the NDs also affects the FL signal enhancement with the variation of the film thickness. For example, the radius of curvature is the same as that of the Fig. 5(c), while the number of positioned NDs was increased to nine [Fig. 5(d)]. The simulated results showed that the increased number of NDs would enhance the FL signal collection. In experiment, we set the laser power to 20 mW and verified the FL collection of the three sensing probes in water. In Fig. 6, it can be seen that the probes with the 9.20 µm, 12.65 µm, and 17.52 µm UV adhesive film coating correspond to the collected FL energy of 11,745 counts, 20,287 counts, and 42,549 counts, respectively. With the increase of UV adhesive thickness, the FL signal energy is significantly enhanced, which is consistent with the simulation results.
Figure 5.Ray tracing simulations are shown in (a)–(d). The simulations are conducted using Optgeo.
Figure 6.Collected FL energy of three probes with different thickness.
Finally, the fabricated fiber-based quantum probe was demonstrated for temperature sensing with an input laser power of 20 mW. The three sensors were placed into a water bath, and the FL signals at the initial temperature of 17°C were recorded. The FL spectra were recorded at intervals of 5°C from 20°C to 75°C. Figure 7(a) shows the temperature response spectral lines of probe III. It can be seen that the zero-phonon line (ZPL) has a significant broadening effect and shifts with temperature variation. The temperature response is 0.034 nm/°C, and it is consistent with the experimental phenomenon in Refs. [7,19]. Nonetheless, it is not enough to obtain the temperature sensitivity of the sensor only by reading the drift information of ZPL, because the too little sensitivity cannot meet the temperature measurement requirements in some specific environments.
Figure 7.(a) Temperature dependent FL signal spectra of probe III; (b) temperature increase and decrease calibration curve of probe III; (c) comparison of temperature increase and decrease of probe III; (d) trend of FL energy attenuation of the three sensors compared at 17°C, 45°C, and 75°C.
However, we found that as the temperature increased the energy of the main excitation peak, ZPL also decreased. This was attributed to the fact that the thermal expansion induced refractive index decrease of the UV adhesive film caused the leakage of excitation light and FL signals. The FL decreasing due to an increase of non-radiative pathways in the high temperature also contributed to the energy decrease of ZPL[
4. Conclusion
In summary, a simple integration method for NDs to fiber has been proposed. The simulated and experimental results showed that the excitation and collection efficiency of the FL signal can be enhanced by increasing the thickness of NDs adhesive coating. In addition, coating the NDs adhesive surface with a metal film will further increase the FL signal of the sensor. In the temperature measurement, for every 1°C increase, the energy of FL of the sensing probe decreases by 174,840 counts. The proposed NDs integration approach paves the way for developing fiber-based NV thermometry and magnetometry.
References
[1] V. Fedotov, S. M. Blakley, E. E. Serebryannikov, P. Hemmer, M. O. Scully, A. M. Zheltikov. High-resolution magnetic field imaging with a nitrogen-vacancy diamond sensor integrated with a photonic-crystal fiber. Opt. Lett., 41, 472(2016).
[2] S. M. Blakley, I. V. Fedotov, S. Y. Kilin, A. M. Zheltikov. Room-temperature magnetic gradiometry with fiber-coupled nitrogen-vacancy centers in diamond. Opt. Lett., 40, 3727(2015).
[3] A. M. Wojciechowski, P. Nakonieczna, M. Mrózek, K. Sycz, A. Kruk, M. Ficek, M. Głowacki, R. Bogdanowicz, W. Gawlik. Optical magnetometry based on nanodiamonds with nitrogen-vacancy color centers. Materials, 12, 2951(2019).
[4] B. W. Zhao, Y. Dong, S. C. Zhang, X. D. Chen, W. Zhu, F. W. Sun. Improving the NV generation efficiency by electron irradiation. Chin. Opt. Lett., 18, 080201(2020).
[5] F. Dolde, H. Fedder, M. W. Doherty, T. Nöbauer, F. Rempp, G. Balasubramanian, T. Wolf, F. Reinhard, L. C. L. Hollenberg, F. Jelezko, J. Wrachtrup. Electric-field sensing using single diamond spins. Nat. Phys., 7, 459(2011).
[6] F. Dolde, M. W. Doherty, J. Michl, T. Nöbauer, F. Rempp, G. Balasubramanian, F. Jakobi, N. B. Manson, J. Wrachtrup. Nanoscale detection of a single fundamental charge in ambient conditions using the NV-center in diamond. Phys. Rev. Lett., 112, 097603(2014).
[7] P. C. Tsai, C. P. Epperla, J. S. Huang, O. Y. Chen, C. C. Wu, H. C. Chang. Measuring nanoscale thermostability of cell membranes with single gold–diamond nanohybrids. Angewandte, 56, 3025(2017).
[8] I. V. Fedotov, S. M. Blakley, E. E. Serebryannikov, N. A. Safronov, V. L. Velichansky, M. O. Scully, A. M. Zheltikov. Fiber-based thermometry using optically detected magnetic resonance. Appl. Phys. Lett., 105, 261109(2014).
[9] S. Bogdanović, S. B. van Dam, C. Bonato, L. C. Coenen, A. J. Zwerver, B. Hensen, M. S. Z. Liddy, T. Fink, A. Reiserer, M. Lončar, R. Hanson. Design and low-temperature characterization of a tunable microcavity for diamond-based quantum networks. Appl. Phys. Lett., 110, 171103(2017).
[10] M. Gregor, R. Henze, T. Schröder, O. Benson. On-demand positioning of a preselected quantum emitter on a fiber-coupled toroidal microresonator. Appl. Phys. Lett., 95, 153110(2009).
[11] S. Bogdanović, M. S. Z. Liddy, S. B. van Dam, L. C. Coenen, T. Fink, M. Lončar, R. Hanson. Robust nano-fabrication of an integrated platform for spin control in a tunable microcavity. APL Photon., 2, 126101(2017).
[12] T. Schröder, A. W. Schell, G. Kewes, T. Aichele, O. Benson. Fiber-integrated diamond-based single photon source. Nano. Lett., 11, 198(2011).
[13] I. V. Fedotov, N. A. Safronov, Y. A. Shandarov, A. A. Lanin, A. B. Fedotov, S. Y. Kilin, K. Sakoda, M. O. Scully, A. M. Zheltikov. Guided-wave-coupled nitrogen vacancies in nanodiamond doped photonic-crystal fibers. Appl. Phys. Lett., 101, 031106(2012).
[14] X. D. Liu, J. M. Cui, F. W. Sun, X. R. Song, F. P. Feng, J. F. Wang, W. Zhu, L. R. Lou, G. Z. Wang. Fiber-integrated diamond-based magnetometer. Appl. Phys. Lett., 103, 143105(2013).
[15] S. M. Blakley, C. Vincent, I. V. Fedotov, X. H. Liu, K. Sower, D. Nodurft, J. Liu, X. H. Liu, V. N. Agafonov, V. A. Davydov, A. V. Akimov, A. M. Zheltikov. Photonic-crystal-fiber quantum probes for high-resolution thermal imaging. Phys. Rev. Appl., 13, 044048(2020).
[16] P. A. Mohammed. Optical and mechanical properties of self-written polymer waveguide between single mode optical fibers using UV photocurable monomer system. Eur. Polym. J., 139, 109950(2020).
[17] K. L. Li, J. B. Du, W. H. Shen, J. C. Liu, Z. Y. He. Improved optical coupling based on a concave cavity lens fabricated by optical fiber facet etching. Chin. Opt. Lett., 19, 050602(2021).
[18] J. P. Tetienne, A. Lombard, D. A. Simpson, C. Ritchie, J. Lu, P. Mulvaney, L. C. L. Hollenberg. Scanning nanospin ensemble microscope for nanoscale magnetic and thermal imaging. Nano. Lett., 16, 326(2016).
[19] B. Dong, C. K. Shi, Z. W. Xu, K. Y. Wang, H. H. Luo, F. W. Sun, P. F. Wang, E. Wu, K. Zhang, J. Y. Liu, Y. Song, Y. X. Fan. Temperature dependence of optical centers in Ib diamond characterized by photoluminescence spectra. Diamond Relat. Mater., 116, 108389(2021).
[20] D. W. Duan, V. K. Kavatamane, S. R. Arumugam, G. Rahane, G. X. Du, Y. K. Tzeng, H. C. Chang, G. Balasubramanian. Laser-induced heating in a high-density ensemble of nitrogen-vacancy centers in diamond and its effects on quantum sensing. Opt. Lett., 44, 2851(2019).
[21] T. Plakhotnik, M. W. Doherty, J. H. Cole, R. Chapman, N. B. Manson. All-optical thermometry and thermal properties of the optically detected spin resonances of the NV-center in nanodiamond. Nano. Lett., 14, 4989(2014).
[22] P. R. Bargo, S. A. Prahl, S. L. Jacques. Collection efficiency of a single optical fiber in turbid media for reflectance spectroscopy. Appl. Opt., 42, 3187(2003).
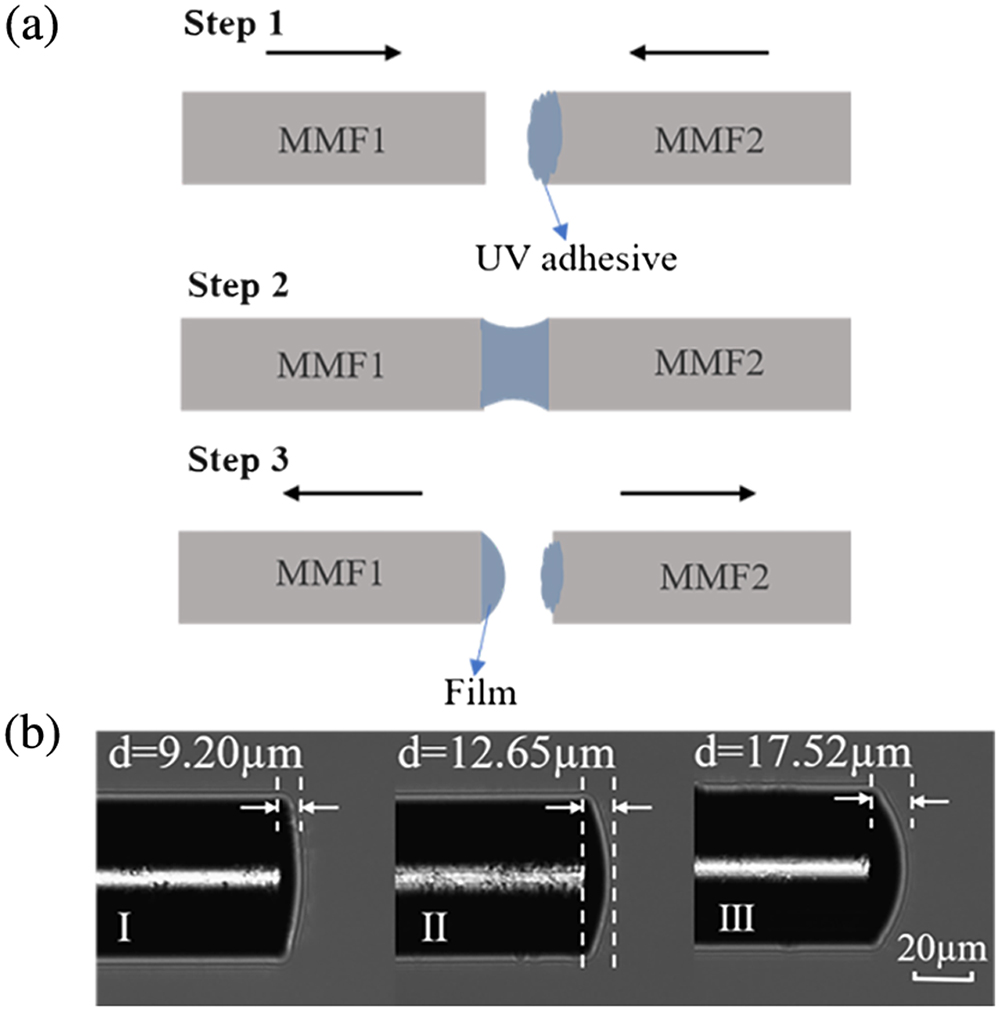
Set citation alerts for the article
Please enter your email address