Abstract
A wavelength-swept fiber laser is proposed and successfully demonstrated based on a bidirectional used linear chirped fiber Bragg grating (LC-FBG). The wavelength-swept operation principle is based on intracavity pulse stretching and compression. The LC-FBG can introduce equivalent positive and negative dispersion simultaneously, which enables a perfect dispersion matching to obtain wide-bandwidth mode-locking. Experimental results demonstrate a wavelength-swept fiber laser that exhibits a sweep rate of about 5.4 MHz over a 2.1 nm range at a center wavelength of 1550 nm. It has the advantages of simple configuration and perfect dispersion matching in the laser cavity.1. INTRODUCTION
Wavelength-swept fiber lasers have attracted much interest due to their wide potential applications in optical coherence tomography (OCT), distributed fiber sensing based on the optical frequency domain reflection (OFDR) technique, continuous wave terahertz generation, and optical communication systems [1–4]. Many technologies have been proposed to build wavelength-swept fiber lasers [5–18]. The most straightforward realization of a swept laser is composed of the gain medium and a tunable bandpass filter in the laser cavity [5,6]. Although the structure is simple, the long build-up time of the new laser signal at each sweeping period intrinsically limits the maximum sweep rate of the laser. In order to significantly improve the sweep rate, Fourier domain mode-locking (FDML) and buffered FDML have been developed [7,8], where the scanning frequency of the tunable filter is matched to the optical round-trip time using a long dispersive shift fiber. By using the FDML scheme, sweep rate has been significantly improved [9,10]. Other methods such as dispersion tuning or dispersion mode-locking techniques [11–13], rapid, -space linear wavelength scanning based on recirculating frequency shifter [14,15] also have been demonstrated, benefiting from the tunable-filter-free configuration. Time-division multiplexing of ultrashort pulses with broad spectrum by incorporation of dispersion outside mode-locked laser sources also were reported [16–18] but with relatively low output power.
Recently, Tozburun et al. demonstrated a rapid dispersion-based wavelength-swept fiber laser [19]. The laser design is based on intracavity pulse stretching and compression, which were, respectively, provided by a 39.394 km single mode fiber (SMF) and a 5.26 km dispersion compensating module. Because it is difficult to make the two lengths of fibers have exactly opposite dispersion, a relative dispersion mismatch would probably exist and degrade the system performance. In this paper, we propose a wavelength-swept fiber laser with perfect dispersion matching during the pulse stretching and compression process. Unlike using two discrete positive and negative dispersive elements, a linear chirped fiber Bragg grating (LC-FBG) is introduced to act as a bidirectional used dispersive unit. Because the group delay responses from the long wavelength end and the short wavelength end of the linear chirped grating are exactly complementary, the implementation of only one chirped grating in the fiber laser has no dispersion mismatch problem. Experimental results demonstrate a wavelength-swept fiber laser that exhibits a sweep rate of about 5.4 MHz over a 2.1 nm range at a center wavelength of 1550 nm. It has the advantages of simple configuration and perfect dispersion matching in the laser cavity.
2. TOPOLOGY AND OPERATION PRINCIPLE
The topology diagram of a wavelength-swept fiber laser based on intracavity pulse stretching and compression is shown in Fig. 1 [19]. An optical amplifier (OA) provides broad bandwidth gain, and an optical bandpass filter (OBF) is used to define the wavelength sweeping range. An intensity modulator is driven by an electrical pulse at a repetition rate equal to a harmonic of the fundamental frequency of the laser cavity to achieve gain switched modulation. Before the oscillation is coupled out of the laser cavity through the output optical coupler (OC), a positive (or negative) dispersive element is used to separate the laser wavelengths in the time domain to obtain wavelength-swept output. To temporally realign the wavelength-swept pulses at the intensity modulator, a negative (or positive) dispersive element with equivalent opposite dispersion to that of the first dispersive element is used before the modulator. In Ref. [19], the positive and negative dispersive elements are implemented by using a few tens of kilometers SMF and dispersion-compensating fiber (DCF), respectively. As stated in the reference, it is a great technical challenge for the dispersion engineering of the laser cavity. Dispersion mismatch will lead to different fundamental frequencies for different wavelengths; consequently, they could not simultaneously mode-lock when they returned to the modulator [20]. This would result in a great reduction in sweeping wavelength range.
Sign up for Photonics Research TOC. Get the latest issue of Photonics Research delivered right to you!Sign up now
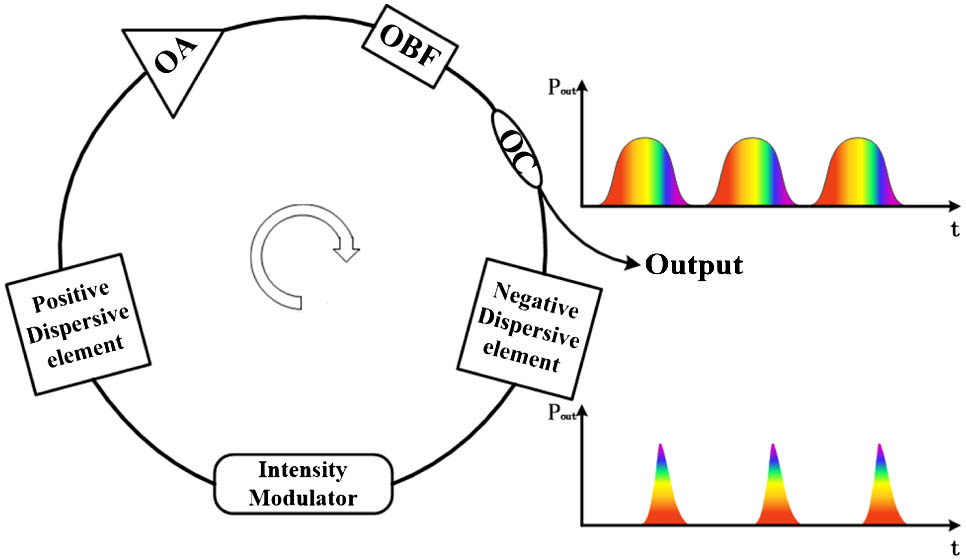
Figure 1.Topology diagram of a wavelength-swept fiber laser based on intracavity pulse stretching and compression.
In order to solve the dispersion mismatch problem, we propose to use an LC-FBG to simultaneously provide positive and negative dispersions, and the topology structure is shown in Fig. 2. Optical pulses of broad bandwidth containing multiple wavelengths from the modulator are sent to the LC-FBG from its short wavelength end (end B) via an optical circulator (CIR-2) so that they can be stretched and separated to obtain a wavelength-swept output due to the dispersion of the LC-FBG. Then, the stretched pulses are compressed when routed to the long wavelength end (end A) of the LC-FBG via CIR-1 to ensure that the wavelengths have the same round-trip time and can be simultaneously mode-locked by the modulator. Because the group delay responses introduced by the bidirectional ends of the LC-FBG are exactly complementary, there will always be perfect dispersion matching for the wavelengths during the process of the pulse stretching and compression. Obviously, the bidirectional use of the LC-FBG has simplified the laser configuration and effectively solved the dispersion mismatch problem in the previously proposed setup.
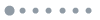
Figure 2.Topology diagram of wavelength-swept fiber laser based on an LC-FBG.
3. EXPERIMENT AND DISCUSSION
Figure 3 shows the experimental setup of the proposed LC-FBG-based wavelength-swept fiber laser. The LC-FBG used in the experiment has a bandwidth of about 2.1 nm at a center wavelength of 1550 nm with a reflectivity of about 100%. The dispersion of the chirped grating is about 816.34 ps/nm, which is comparable with that of 48 km SMF. The measured reflection and transmission spectra of the chirped grating are shown in Fig. 4. Two CIRs were inserted at the two ends of the chirped grating to route the light path and also functioned as optical isolators to ensure unidirectional operation in the laser cavity. An erbium-doped fiber amplifier (EDFA) (Amonics AEDFA-33-B-FA) with more than 30 dB gain was used as broadband OA, followed by an optical tunable bandpass filter (OTBF) (Santec OTF-350) whose bandpass can be tuned to be consistent with the reflection bandwidth of the chirped grating. An intensity modulation (IM) (JDS Uniphase SN-338292M) with an extinction ratio up to 34 dB was driven by a digital delay generator (DDG) (Stanford Research System DG645). A semiconductor optical amplifier (SOA) (CIP SOA-S-OEC-1550) was introduced after the IM to further compensate the system loss. Two polarization controllers (PCs) were placed before the SOA and IM, respectively, to optimize the gain performance and modulation efficiency. The wavelength-swept output was coupled out through the 10% output port of a 90:10 OC-1 and was further divided into two parts by a 50:50 OC-2, with one sent to an oscilloscope (OSC) (Rigol MSO2302A, 300 MHz) after detection by a photodetector (PD) and the other sent to an optical spectrum analyzer (OSA) (Yokogawa AQ6370D) with a resolution of 0.02 nm, respectively, for the time domain and optical domain performance monitoring.
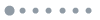
Figure 3.Schematic of the proposed fiber laser based on an LC-FBG.
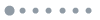
Figure 4.(a) Reflection spectrum and (b) the transmission spectrum of the LC-FBG.
In experiment, the total length of the cavity was about 38 m, and the fundamental frequency was about 5.4 MHz. The DDG was set at a pulse repetition rate of 5.4003 MHz to drive the IM. PC-2 was adjusted to obtain the best modulation. The measured optical spectrum and the waveform of the laser output are shown in Fig. 5. Figure 5(a) shows that the swept wavelength range is about 2.1 nm, limited by the bandwidth of the chirped grating. Figure 5(b) shows the output pulse train, and a detailed waveform is shown in the inset. The pulse repetition rate is 5.4003 MHz, with a pulse width of about 5.4 ns. The output radio frequency (RF) spectrum was measured, and the result is shown in Fig. 6. It can be seen that the side-mode suppression ratio (SMSR) of the RF spectrum is around 50 dB, indicating an excellent active mode-locking status of the laser.
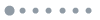
Figure 5.(a) Wide spectrum at the laser output of OC-1. (b) Output pulse train with a detailed waveform in the inset.
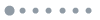
Figure 6.RF spectrum at the laser output of OC-1.
In order to investigate the function of the chirped grating in the laser cavity, the output port was moved to the location , as shown in Fig. 3. Figure 7 shows the pulse train with a detailed pulse waveform in the inset. The pulse width is about 3.6 ns, which has been obviously compressed by the chirped grating compared with the output from OC-1. It indicated that the output from OC-1 was dispersion stretched and the wavelengths had been separated to some extent. It also verified the concept that a bidirectional operated chirped grating can act as the positive and negative dispersion elements to stretch and compress the pulses.
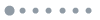
Figure 7.Output pulse train with a detailed waveform in the inset from output port .
To further confirm that the fiber laser is under wavelength-swept operation, another measurement was done. Another OTF was connected to the coupler OC-1, whose center wavelength switched from 1549.72 to 1550.72 nm with a wavelength step of 0.2 nm. The measured spectra and pulse waveforms during the switching process are shown in Figs. 8(a) and 8(b), respectively. It can be seen that different wavelengths correspond to different pulse position in the time domain, and the optical spectrum and the corresponding temporal pulse had an inversed mapping relationship (according to a positive dispersion from the point B of the LC-FBG), which showed a wavelength sweeping operation of the fiber laser.
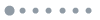
Figure 8.(a) Spectra and (b) pulse waveforms of the laser output for six wavelengths switched from 1549.72 to 1550.72 nm.
It should be noted that the laser sweeping wavelength range is limited by the bandwidth of the LC-FBG used in this experiment. The wavelength-swept range can be widened by using a chirped grating with larger bandwidth of more than 30 nm [21]. Due to the unavailability of such FBG in our laboratory, we simply propose this new concept of bidirectional used LC-FBG in wavelength-swept fiber laser to provide perfect dispersion matching in the laser cavity. Note that long LC-FBG with a large dispersion coefficient may have some nonlinearity and ripples in the group delay, which may make them unsuitable for high-performance applications. But the characteristics of the LC-FBG can be optimized by proper apodization method [22]. In addition, a cascaded LC-FBG structure possibly can be used, though it may require additional study.
Another point we have to note is that the net dispersion of the laser cavity is very small due to the SOA having opposite dispersion to that of SMF in the C band [23]. Thus, the nonzero dispersion would not be a significant factor compared with the dispersion mismatch introduced by the DCF and SMF in Ref. [19].
In experiments, sweeping from a longer wavelength to a shorter wavelength was obtained. Forward sweeping from shorter wavelengths to longer wavelengths easily can be achieved by changing the direction of the LC-FBG.
4. CONCLUSION
In conclusion, a novel wavelength-swept laser based on LC-FBG is proposed and experimentally demonstrated. The chirped grating is used as a bidirectional dispersive unit, which provides exact complementary group delay responses, so that the stretched pulse sequence can be compressed to original status without any dispersion mismatch. The perfect dispersion matching helps to obtain wide bandwidth mode-locking of the laser. The proposed fiber laser has the advantage of less system complexity and perfect dispersion matching in the laser cavity.
References
[1] T. Klein, W. Wieser, L. Reznicek, A. Neubauer, A. Kampik, R. Huber. Multi-MHz retinal OCT. Biomed. Opt. Express, 4, 1890-1908(2013).
[2] C. Y. Ryu, C. S. Hong. Development of fiber Bragg grating sensor system using wavelength-swept fiber laser. Smart Mater. Struct., 11, 468-473(2002).
[3] D. P. Zhou, Z. G. Qin, W. H. Li, L. Chen, X. Y. Bao. Distributed vibration sensing with time-resolved optical frequency-domain reflectometry. Opt. Express, 20, 13138-13145(2012).
[4] S. S. Jyu, S. F. Liu, W. W. Hsiang, Y. Lai. Fiber dispersion measurement with a swept-wavelength pulse light source. IEEE Photon. Technol. Lett., 22, 598-600(2010).
[5] S. H. Yun, C. Boudoux, G. J. Tearney, B. E. Bouma. High-speed wavelength-swept semiconductor laser with a polygon-scanner-based wavelength filter. Opt. Lett., 28, 1981-1983(2003).
[6] R. Huber, M. Wojtkowski, K. Taira, J. G. Fujimoto, K. Hsu. Amplified, frequency swept lasers for frequency domain reflectometry and OCT imaging: design and scaling principles. Opt. Express, 13, 3513-3528(2005).
[7] R. Huber, M. Wojtkowski, J. G. Fujimoto. Fourier domain mode locking (FDML): A new laser operating regime and applications for optical coherence tomography. Opt. Express, 14, 3225-3237(2006).
[8] R. Huber, D. C. Adler, J. G. Fujimoto. Buffered Fourier domain mode locking: unidirectional swept laser sources for optical coherence tomography imaging at 370,000 lines/s. Opt. Lett., 31, 2975-2977(2006).
[9] M. Y. Jeon, J. Zhang, Q. Wang, Z. P. Chen. High-speed and wide bandwidth Fourier domain mode-locked wavelength-swept laser with multiple SOAs. Opt. Express, 16, 2547-2554(2008).
[10] W. Wieser, T. Klein, D. C. Adler, F. Trepanier, C. M. Eigenwillig, S. Karpf, J. M. Schmitt, R. Huber. Extended coherence length megahertz FDML and its application for anterior segment imaging. Biomed. Opt. Express, 3, 2647-2657(2012).
[11] S. Yamashita, M. Asano. Wide and fast wavelength-tunable mode-locked fiber laser based on dispersion tuning. Opt. Express, 14, 9299-9306(2006).
[12] Y. Takubo, S. Yamashita. In vivo OCT imaging using wavelength-swept fiber laser based on dispersion tuning. IEEE Photon. Technol. Lett., 24, 979-981(2012).
[13] Y. Takubo, S. Yamashita. High-speed dispersion-tuned wavelength-swept fiber laser using a reflective SOA and a chirped FBG. Opt. Express, 21, 5130-5139(2013).
[14] M. G. Wan, L. Wang, F. Li, Y. Cao, X. D. Wang, X. H. Feng, B. O. Guan, P. K. A. Wai. Rapid, k-space linear wavelength scanning laser source based on recirculating frequency shifter. Opt. Express, 24, 27614-27621(2016).
[15] G. Gavioli, E. Torrengo, G. Bosco, A. Carena, S. Savory, F. Forghieri, P. Poggiolini. Ultra-narrow-spacing 10-channel 1.12 Tb/s D-WDM long-haul transmission over uncompensated SMF and NZDSF. IEEE Photon. Technol. Lett., 22, 1419-1421(2010).
[16] S. Moon, D. Y. Kim. Ultra-high-speed optical coherence tomography with a stretched pulse supercontinuum source. Opt. Express, 14, 11575-11584(2006).
[17] J. Xu, C. Zhang, J. Xu, K. K. Wong, K. K. Tsia. Megahertz all-optical swept-source optical coherence tomography based on broadband amplified optical time-stretch. Opt. Lett., 39, 622-625(2014).
[18] T. J. Ahn, Y. Park, J. Azana. Ultrarapid optical frequency-domain reflectometry based upon dispersion-induced time stretching: principle and applications. IEEE J. Sel. Top. Quantum, 18, 148-165(2012).
[19] S. Tozburun, M. Siddiqui, B. J. Vakoc. A rapid, dispersion-based wavelength-stepped and wavelength-swept laser for optical coherence tomography. Opt. Express, 22, 3414-3424(2014).
[20] K. Chan, C. Shu. Compensated dispersion tuning in harmonically mode-locked fiber laser. Appl. Phys. Lett., 75, 891-893(1999).
[21] J. F. Brennan, E. Hernadez, J. A. Valenti, P. G. Sinha, M. R. Matthews, D. E. Elder, G. A. Beauchesne, C. H. Byrd. Wide-bandwidth chirped fiber Bragg gratings with low delay ripple amplitude. U.S. patent(2004).
[22] K. Ennser, M. N. Zervas, R. I. Laming. Optimization of apodized linearly chirped fiber gratings for optical communications. IEEE J. Quantum Electron., 34, 770-778(1998).
[23] Z. Wang, Q. Lin, Y. T. Jian, L. L. Liu, C. Q. Wu. Dispersion measurement of the semiconductor optical amplifiers. Proc. SPIE, 9233, 92331J(2014).