
- Chinese Optics Letters
- Vol. 19, Issue 12, 121401 (2021)
Abstract
1. Introduction
In a wavelength-division multiplexing (WDM) system, optical data signals are transmitted in parallel wavelength channels, significantly enhancing the communication capacity and spectrum efficiency of optic fibers[
Figure 1.Principles of OIL-based low-noise amplification of DKS microcomb lines. (a) Conventional multi-wavelength source consists of a large number of discrete lasers showing severe random frequency drift; (b) DKS microcomb generated by an external cavity laser diode (ECDL) amplified by using an EDFA, but at the sacrifice of OSNR degradation; (c) OIL-laser-based DKS microcomb amplification scheme provides simultaneous low noise and high gain. (d) A conceptual image of an integrated chip-scale optical data transmitter using DKS comb lines as WDM data carriers, which are power boosted using the OIL laser scheme.
When pumped by a continuous-wave laser, the DKS microcomb can be produced in a high-quality (Q)-factor microcavity relying on a double balance between parametric gain and loss, as well as dispersion and Kerr nonlinearity.
Nowadays, each DKS microcomb can offer hundreds of evenly spaced laser lines with frequency spacing ranging from gigahertz (GHz) to terahertz (THz), while maintaining extremely high mutual coherence among all comb lines[
Sign up for Chinese Optics Letters TOC. Get the latest issue of Chinese Optics Letters delivered right to you!Sign up now
However, the generating dynamics of the DKS microcomb usually suffer from quite low conversion efficiency from the incident pump laser to the generated comb lines[
We note that the technique of an optical injection locking (OIL) laser can be a candidate method for power amplification and equalization of DKS microcomb lines[
In this work, for the first time, to the best of our knowledge, we demonstrate low-noise power amplification and equalization of DKS microcomb lines via OIL of low-cost distributed feedback (DFB) lasers. The optical power of DKS microcomb lines can be amplified by 30 dB (from
2. Results and Discussion
Figure 2(a) shows the detailed setup of our experiment. In our experiment, a high-Q WGM micro-rod is employed as the Kerr nonlinear cavity, which is fabricated by
Figure 2.Characterization of a DKS microcomb generated in a WGM microcavity. (a) Experimental setup for DKS microcomb generation, linewidth measurement, laser injection locking, and coherent data transmission. (b) Microscopy image of WGM micro-rod resonator used in experiment. (c) Optical spectrum of a single-DKS comb state generated in a WGM micro-resonator. (d) Measured linewidths of 20 DKS comb lines adjacent to the pump laser. (e) Self-heterodyne interferometer beat note spectrum measured for the exemplified comb lines #5 and #10.
Importantly, the total output optical power of the DKS microcomb is about
Next, we experimentally investigate OIL-enabled amplification and equalization of the generated DKS comb lines. As previously reported, OIL of a semiconductor DFB laser can be achieved at an injection ratio as low as
Figure 3.Characterization of OIL-based DKS comb line amplification. (a), (b) Measured optical spectra of initial DKS comb lines and slave lasers after OIL. (c) Comparison of the optical spectra between OIL slave lasers and DKS comb lines amplified by two-stage EDFAs. (d), (e) Measured linewidth beat note RF spectra of free-running and OIL slave lasers by using the delayed self-heterodyne interferometer method. (f) Comparison of the beat note between two adjacent DKS micro-comb lines before and after OIL.
Furthermore, as shown in Figs. 3(d) and 3(e), the linewidths of free-running slave lasers and injection locked slave lasers are also measured. It is seen that the slave laser linewidths are significantly reduced from about 300 kHz to about 2.5 kHz, consistent with the linewidths of the corresponding master soliton comb lines [see Fig. 2(d)]. Also, as mentioned above, mutual frequency and phase coherence among DKS comb lines can be imposed onto OIL slave lasers, so that the guard bands that deal with the random frequency drifts suffered by independent laser carriers are minimized, or even support seamless super channel stitching[
Next, we demonstrate coherent optical communication by using the power enhanced DKS microcomb lines based on OIL lasers. At the transmitter side, two slave DFB lasers injection-locked by the corresponding DKS comb lines are adopted as the carrier tones and encoded with 18.75 Gbaud 16-quadrature amplitude modulation (QAM) coherent orthogonal optical frequency division multiplexing (CO-OFDM) data signals within a commercial IQ modulator, driven by an electrical arbitrary waveform generator (eAWG) operating at 25 GSa/s. The CO-OFDM symbol is from a 128-point fast Fourier transformation (FFT); 96 subcarriers are used to carry the pilot and data information with the cyclic prefix being 1/16 of the total FFT length. The 16-QAM CO-OFDM signal is transmitted through 100 km standard single-mode fiber. At the receiver side, we use a tunable DFB laser as the local oscillator (LO) to implement coherent data detection within a 30 GHz IQ receiver. The arrived power of each signal channel is about
Figure 4.Comparison between the OIL-based low-noise amplification scheme and conventional EDFA scheme. The constellation diagrams of the received data plotted with blue represent the OIL scheme, and red is for the EDFA scheme. The BER for each channel is listed under each constellation diagram.
3. Conclusion
In conclusion, we proposed and demonstrated high-performance DKS comb line power amplification by using the OIL technique. Superior gain factor larger than 35 dB and OSNR performance as high as 60 dB are obtained by injection locking for two DFB lasers using comb lines from a 20 GHz spaced DKS microcomb. Also, thanks to the photon-photon interaction nature of OIL, the superior merits of DKS comb lines, including high-frequency stability, mutual phase coherence, and narrow linewidth, are imposed onto the slave DFB lases. Furthermore, we also demonstrated coherent optical data transmission by adopting the OIL-based method to amplify DKS comb lines and use them as a data carrier, and prominently better performance is obtained from comb power lift via the traditional EDFA-based amplification scheme, confirming the applicability of the proposal. In the present proof-of-concept experiment, we used bulk DFB lasers and passive devices (Mux/Demux, splitter, etc.). However, each of these key modules can find its solution for photonics integration[
References
[1] P. J. Winzer, D. T. Neilson, A. R. Chraplyvy. Fiber-optic transmission and networking: the previous 20 and the next 20 years. Opt. Express, 26, 24190(2018).
[2] X. Liu, S. Chandrasekhar, P. J. Winzer. Digital signal processing techniques enabling multi-Tb/s superchannel transmission: an overview of recent advances in DSP-enabled superchannels. IEEE Signal Process. Mag., 31, 16(2014).
[3] P. M. Palomo, J. N. Kemal, M. Karpov, A. Kordts, J. Pfeifle, M. H. P. Pfeiffer, P. Trocha, S. Wolf, V. Brasch, M. H. Anderson, R. Rosenberger, K. Vijayan, W. Freude, T. J. Kippenkerg, C. Koos. Microresonator-based solitons for massively parallel coherent optical communications. Nature, 546, 274(2017).
[4] B. Corcoran, M. Tan, X. Xu, A. Boes, J. Wu, T. G. Nguyen, S. T. Chu, B. E. Little, R. Morandotti, A. Mitchell, D. J. Moss. Ultra-dense optical data transmission over standard fibre with a single chip source. Nat. Commun., 11, 2568(2020).
[5] Y. Geng, X. Huang, W. Cui, Y. Ling, B. Wu, J. Zhang, X. Yi, B. Wu, S.-W. Huang, K. Qiu, C. W. Wong, H. Zhou. Terabit optical OFDM superchannel transmission via coherent carriers of a hybrid chip-scale soliton frequency comb. Opt. Lett., 43, 2406(2018).
[6] T. Herr, V. Brasch, J. D. Jost, C. Y. Wang, N. M. Kondratiev, M. L. Gorodetsky, T. J. Kippenberg. Temporal solitons in optical microresonators. Nat. Photon., 8, 145(2014).
[7] V. Brasch, M. Geiselmann, T. Herr, G. Lihachev, M. H. Pfeiffer, M. L. Gorodetsky, T. J. Kippenberg. Photonic chip-based optical frequency comb using soliton Cherenkov radiation. Science, 351, 357(2016).
[8] C. Bao, L. Zhang, A. Matsko, Y. Yan, Z. Zhao, G. Xie, A. M. Agarwal, L. C. Kimerling, J. Michel, L. Maleki, A. E. Willner. Nonlinear conversion efficiency in Kerr frequency comb generation. Opt. Lett., 39, 6126(2014).
[9] Q. Wen, J. Qin, W. Cui, Y. Geng, H. Zhou, K. Qiu. Increasing the power of dissipative Kerr soliton microcomb by bus-resonator coupling control. Asia Communications and Photonics Conference, T4D-5(2020).
[10] Z. Liu, R. Slavík. Optical injection locking: from principle to applications. J. Lightwave Technol., 38, 43(2020).
[11] J. Wang, T. Jia, S. Wang, T. Li, C. Ma, T. Xie, Y. Yu, J. Yu. Wavelength synchronization technology for UDWDM-PON transmitter based on injection locking. Chin. Opt. Lett., 19, 010602(2021).
[12] H. Guo, M. Karpov, E. Lucas, A. Kordts, M. H. Pfeiffer, V. Brasch, G. Lihachev, V. E. Lobanov, M. L. Gorodetsky, T. J. Kippenberg. Universal dynamics and deterministic switching of dissipative Kerr solitons in optical microresonators. Nat. Phys., 13, 94(2017).
[13] P. Del’Haye, S. A. Diddams, S. B. Papp. Laser-machined ultra-high-Q microrod resonators for nonlinear optics. Appl. Phys. Lett., 102, 221119(2013).
[14] Q. Wen, W. Cui, Y. Geng, H. Zhou, K. Qiu. Precise control of micro-rod resonator free spectral range via iterative laser annealing. Chin. Opt. Lett., 19, 071903(2021).
[15] H. Zhou, Y. Geng, W. Cui, S. Huang, Q. Zhou, K. Qiu, C. W. Wong. Soliton bursts and deterministic dissipative Kerr soliton generation in auxiliary-assisted microcavities. Light: Sci. Appl., 8, 50(2019).
[16] N. Park, J. W. Dawson, K. J. Vahala. Linewidth and frequency jitter measurement of an erbium-doped fiber ring laser by using a loss-compensated, delayed self-heterodyne interferometer. Opt. Lett., 17, 1274(1992).
[17] A. V. Cherenkov, V. E. Lobanov, M. L. Gorodetsky. Dissipative Kerr solitons and Cherenkov radiation in optical microresonators with third-order dispersion. Phys. Rev. A, 95, 033810(2017).
[18] W. Liang, D. Eliyahu, V. S. Ilchenko, A. A. Savchenkov, A. B. Matsko, D. Seidel, L. Maleki. High spectral purity Kerr frequency comb radio frequency photonic oscillator. Nat. Commun., 6, 7957(2015).
[19] J. Liu, E. Lucas, A. S. Raja, J. He, J. Riemensberger, R. N. Wang, M. Karpov, H. Guo, R. Bouchand, T. J. Kippenberg. Photonic microwave generation in the X- and K-band using integrated soliton microcombs. Nat. Photon., 14, 486(2020).
[20] J. R. Stone, T. C. Briles, T. E. Drake, D. T. Spencer, D. R. Carlson, S. A. Diddams, S. B. Papp. Thermal and nonlinear dissipative-soliton dynamics in Kerr-microresonator frequency combs. Phys. Rev. Lett., 121, 063902(2018).
[21] X. Yi, Q. F. Yang, X. Zhang, K. Y. Yang, X. Li, K. Vahala. Single-mode dispersive waves and soliton microcomb dynamics. Nat. Commun., 8, 14869(2017).
[22] R. Kakarla, J. Schröder, P. A. Andrekson. Optical injection locking at sub nano-watt powers. Opt. Lett., 43, 5769(2018).
[23] Y. Doumbia, T. Malica, D. Wolfersberger, K. Panajotov, M. Sciamanna. Optical injection dynamics of frequency combs. Opt. Lett., 45, 435(2020).
[24] T. Komljenovic, D. Huang, P. Pintus, M. A. Tran, M. L. Davenport, J. E. Bowers. Photonic integrated circuits using heterogeneous integration on silicon. Proc. IEEE, 106, 2246(2018).
[25] M. R. Billah, M. Blaicher, T. Hoose, P.-I. Dietrich, P. Marin-Palomo, N. Lindenmann, A. Nesic, A. Hofmann, U. Troppenz, M. Moehrle, S. Randel, W. Freude, C. Koos. Hybrid integration of silicon photonics circuits and InP lasers by photonic wire bonding. Optica, 5, 876(2018).
[26] Z. Zhang, J. Hu, H. Chen, F. Li, L. Zhao, J. Gui, Q. Fang. Low-crosstalk silicon photonics arrayed waveguide grating. Chin. Opt. Lett., 15, 041301(2017).
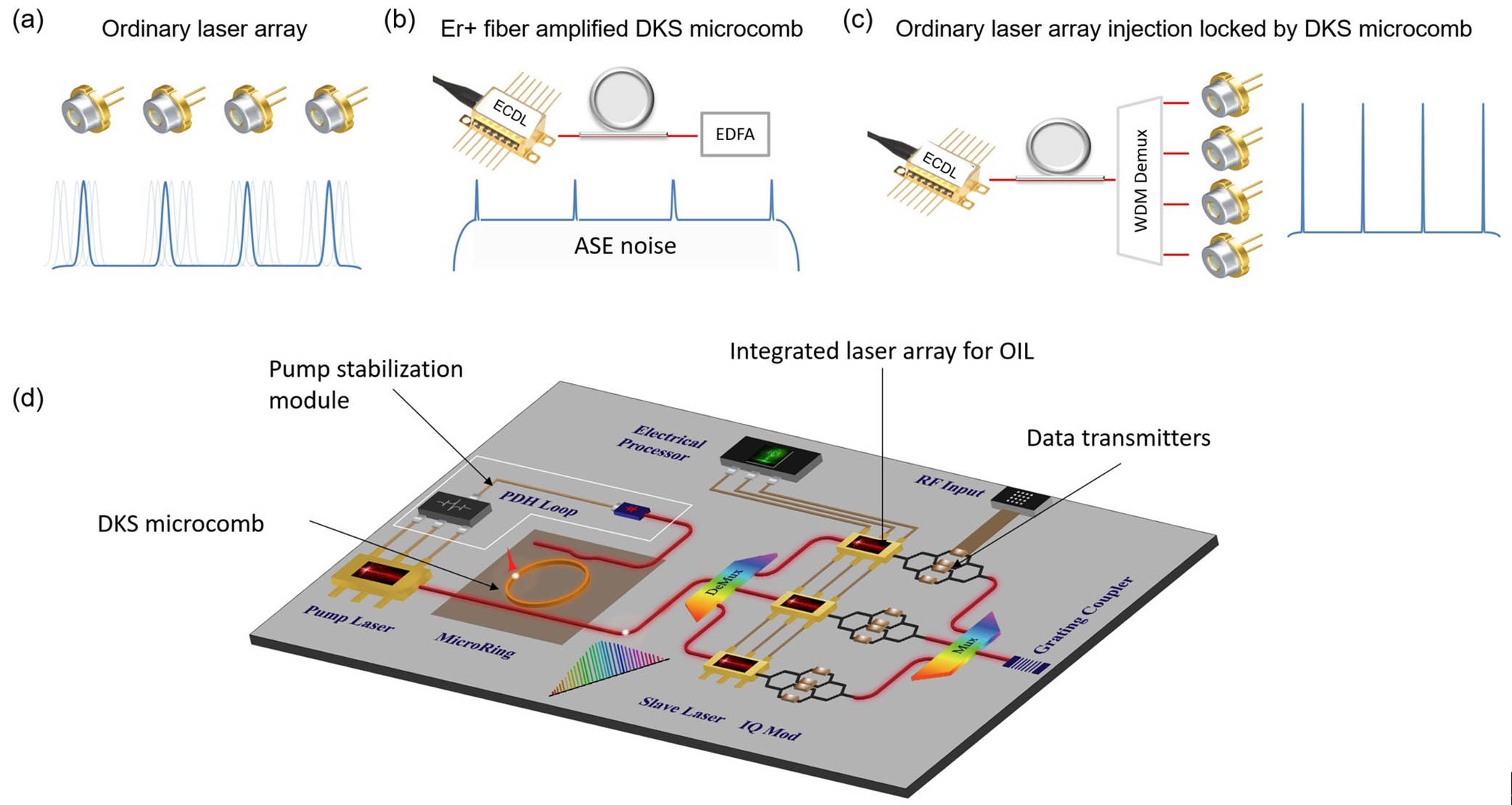
Set citation alerts for the article
Please enter your email address