Abstract
Surface-wave-based optical sensing of an analyte in a fluid relies on the sensitivity of the surface wave to the electromagnetic properties of the analyte-containing fluid in the vicinity of the guiding interface. Surfaceplasmon-polariton (SPP) waves are most commonly used for optical sensing because of the ease of the excitation of an SPP wave when the fluid is partnered with a metal. If the fluid is replaced by a porous, anisotropic, and periodically nonhomogeneous solid filled with the fluid, while the metal is replaced by an isotropic homogeneous dielectric material, the surface wave is called a Dyakonov–Tamm (DT) wave. We have theoretically determined that the incorporation of the DT-waveguiding interface in a prism-coupled configuration provides an alternative to the analogous SPP wave-based sensor, with comparable dynamic sensitivity.1. INTRODUCTION
Any electromagnetic surface wave propagates bound to an interface of two dissimilar materials [1,2]. This localization of the surface wave to the interface allows its use in optical sensing, because a small change in the electromagnetic properties of either of the two partnering materials near the interface can result in a significant change in the characteristics of the surface wave.
Surface-plasmon-polariton (SPP) waves are extensively used for optical sensors [3–7]. The propagation of an SPP wave is guided by the interface of a metal and a dielectric material. Although the partnering dielectric material is usually a fluid containing the analyte to be sensed [3–6], it can also be a porous material that is infiltrated by the analyte-containing fluid [8]. Interestingly, the experimentally measured dynamic sensitivity of a prism/metal/fluid-infiltrated porous material/fluid setup [8] has been found to significantly exceed the theoretical sensitivity of the prism/metal/fluid setup [4].
A surface wave called the Tamm wave is guided by the interface of two isotropic dielectric materials, at least one of which is periodically nonhomogeneous in the direction perpendicular to the interface [9,10]. The Tamm wave has also been experimentally exploited for optical sensing, with the analyte-containing fluid partnering a periodically stratified solid material [11–13].
Sign up for Photonics Research TOC. Get the latest issue of Photonics Research delivered right to you!Sign up now
Surface waves called Dyakonov–Tamm (DT) waves were theoretically predicted a few years ago [14]. Guided by the interface of two dielectric materials of which at least one is both anisotropic and periodically nonhomogeneous normal to the interface [2], DT waves were experimentally observed very recently [15,16].
Not surprisingly, DT waves should also be useful for optical sensing. In the initial proposal [17], the commonplace prism-coupled configuration [2,5,6] was adopted. A monochromatic collimated light beam is supposed to be incident on one of the two slanted faces of a prism made of a material with a sufficiently high refractive index . The base of the prism is coated with a chiral sculptured thin film (STF) [18], which is a porous, anisotropic, and periodically nonhomogeneous material. When the chiral STF contacts the analyte-containing fluid, their interface can guide a DT wave [17]. The chiral STF functions as an anisotropic and periodically nonhomogeneous partner, while the fluid is the isotropic and homogeneous partner. The intensity of light exiting the second slanted face of the prism is recorded as the angle of incidence on the base of the prism is varied by changing the direction of propagation of the light beam incident on the first slanted face of the prism. A pronounced and rather narrow dip in the recorded intensity can indicate the excitation of a DT wave.
As the analyte-containing fluid also infiltrates the chiral STF due to its porosity, the proposed sensor should be quite sensitive as changes in the concentration of the analyte will affect the dielectric properties of both partnering materials. However, this sensing modality does not offer flexibility in the choice of the partnering isotropic dielectric material.
In this paper, we focus our attention on a new sensing modality exploiting the DT-wave phenomenon. As shown in Fig. 1(a), a nonporous layer of thickness and made of an isotropic material of refractive index is interposed between the prism and the chiral STF. With a proper choice of , it would be possible to excite a DT wave guided by the interface of the nonporous solid material and the fluid-infiltrated chiral STF, rather than the interface of the fluid-infitrated chiral STF and the fluid. A short description of the model used for the fluid-infiltrated chiral STF is presented in Section 2 and the numerical results are presented and discussed in Section 3. Concluding remarks are presented in Section 4.
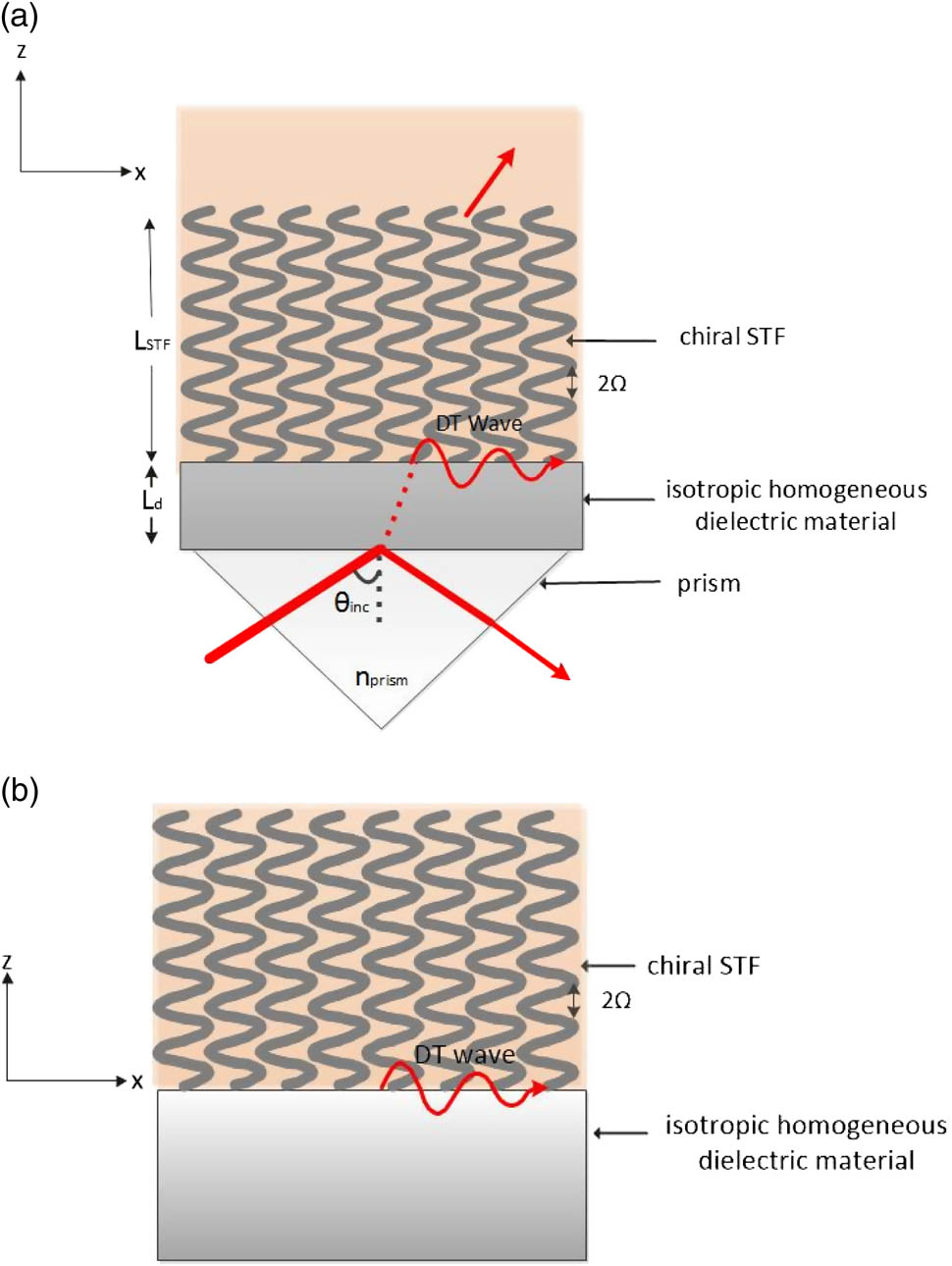
Figure 1.Schematic representations: (a) proposed optical-sensing modality, and (b) underlying canonical boundary-value problem.
2. FLUID-INFILTRATED CHIRAL STF
A schematic of the canonical boundary-value problem underlying the sensing modality proposed in this paper is shown in Fig. 1(b). The half space is occupied by the isotropic partner of refractive index , and the half-space is occupied by the chiral STF infiltrated with an analyte-containing fluid of refractive index . A change in analyte concentration would alter .
Essentially, a chiral STF is fabricated by obliquely directing a collimated vapor flux under low pressure onto a uniformly rotating substrate [18]. The angle between the direction of the vapor flux and the substrate is denoted by . Under suitable conditions, an assembly of parallel nanohelixes form, with the helical axes perpendicular to the substrate. The porosity and morphology of a chiral STF can be tailored during fabrication [18].
The relative permittivity dyadic of a chiral STF can be stated as [18] where the superscript denotes the transpose; the dyadic involves as the helical pitch and either for structural right-handedness or for structural left-handedness; and the dyadic contains as the angle of rise of the nanohelixes. Both and the scalars , , and depend on the angle as well as on the evaporated material. Furthermore, also depend on the free-space wavelength and . Each nanohelix can be viewed as a string of highly elongated ellipsoidal inclusions, which permits the use of homogenization theory to predict the effective relative permittivity dyadic of a fluid-infiltrated chiral STF from the effective relative permittivity dyadic of an uninfiltrated chiral STF [2,19].
3. ILLUSTRATIVE NUMERICAL RESULTS AND DISCUSSION
For all numerical results presented here, we fixed , , , and . We chose magnesium flouride ( [15]) as the isotropic dielectric partner. We used the forward and inverse Bruggeman homogenization formalisms in the manner detailed elsewhere [19] to estimate for the chiral STF infiltrated with a fluid of refractive index by taking measured data for a columnar thin film made by evaporating patinal titanium oxide [20]. Also, consistently with predecessor works [14,17,19], we used ; furthermore, we fixed , , and when .
Let us begin with the canonical boundary-value problem depicted in Fig. 1(b), because it provides predictions for the prism-coupled configuration of Fig. 1(a). Without significant loss of generality and for the sake of illustration, we let the DT wave propagate along the axis with an dependence. Adopting the theoretical formalism described elsewhere [2,14] for the dependence on , we obtained a dispersion equation for the relative wavenumber , where is the free-space wavenumber. The computed values of are presented in Fig. 2 against the refractive index of the fluid infiltrating the void regions of the chiral STF.
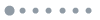
Figure 2.Values of relative wavenumbers of DT waves guided by the interface of magnesium fluoride () and the chosen titanium-oxide chiral STF infiltrated by a fluid of refractive index . In Figs. 2–4, the blue and red curves represent different DT waves.
For a specific , the chosen interface supports either one or two DT waves, represented by the blue and red curves in Fig. 2. Both of these waves have different phase speeds, spatial profiles, and degree of localization to the interface between the partnering materials. No polarization state can be assigned to either of the two DT waves, because of the rotational nonhomogeneity of the chiral STF (i.e., Section 1.7.4 of [2]).
In Fig. 2, is purely real. If the dielectric material of refractive index were to be replaced by a metal, the DT waves would be replaced by SPP waves with complex [2,19]. SPP waves attenuate during propagation, but ideally DT waves do not.
In the prism-coupled configuration, a DT wave is excited when the absorptance as a function of the incidence angle (1) has a peak that is independent of the thicknesses of the partnering dielectric materials beyond threshold values, and (2) the absorptance peak’s angular position is the same as the prediction from the underlying canonical boundary-value problem [2,14]. Figure 3 shows predicted as a function of . All of the values of are practically convenient as they lie between 30° and 50°.
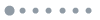
Figure 3.Values of the angle of incidence in the prism-coupled configuration in relation to the refractive index of the fluid infiltrating the chiral STF, as predicted by the canonical boundary-value problem.
The dynamic sensitivity is the change in the angular position of the absorptance peak in the prism-coupled configuration due to change in the refractive index of the fluid infiltrating the chiral STF. The predicted values of versus are presented in Fig. 4. A comparison of the sensitivity of DT waves in Fig. 4 with that of SPP waves using the same prism and the same chiral STF [19] indicates that the sensitivity of DT waves is generally similar to that of SPP waves. For the SPP waves, by using aluminum instead of magnesium fluoride, lies between 22° and 32° per refractive index unit (RIU). For the DT waves studied in this paper, lies between 14° and 27° per RIU.
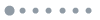
Figure 4.Dynamic sensitivity as a function of in the prism-coupled configuration computed from the predicted data presented in Fig. 3.
Let us now present the actual results of the prism-coupled configuration, the algorithm for the computations being explained in detail elsewhere [2]. The light incident on the base of the prism is assumed to have an electric field of unit magnitude. For , there can be no transmittance in the fluid on top of the fluid-infitrated chiral STF in Fig. 1(a), so that the absorptance and the reflectance (calculated at the base of the prism) must add up to unity. In the plots of absorptance versus the incidence angle , the excitation of a DT wave is indicated by a peak that is independent of the thicknesses of the partnering dielectric materials beyond threshold values. The thickness-dependent peaks could indicate the excitation of waveguide modes [8,21].
Figure 5 presents the absorptances and for incident - and -polarized light, respectively, when , , , and . For these calculations, we set . The tiny imaginary part was added to get nonzero values of the absorptances [17].
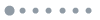
Figure 5.Absorptances (a) and (b) plotted as functions of for , when , , , and . Downward arrows indicate the peaks that represent the excitation of DT waves.
When , there can be no transmission for ; furthermore, Fig. 3 indicates that the canonical boundary-value problem predicts the excitation of one DT wave at in the prism-coupled configuration. In Fig. 5(a), the -peak at is independent of . The plots of in Fig. 5(b) show that the DT wave at can be excited by -polarized incident plane waves as well. We have verified that these conclusions also hold for nm. Accordingly, we conclude that linearly polarized light can excite DT waves and sense the refractive index variation.
4. CONCLUDING REMARKS
Our numerical studies have demonstrated that the excitation of DT waves guided by the planar interface of an isotropic homogeneous dielectric material and a porous chiral STF could be used for optical sensing, with comparable sensitivity as that of the SPP-waves-based sensors with the same prism and chiral STF [19].
References
[1] A. D. Boardman. Electromagnetic Surface Modes(1982).
[2] J. A. Polo, T. G. Mackay, A. Lakhtakia. Electromagnetic Surface Waves: A Modern Perspective(2013).
[3] R. J. Green, R. A. Frazier, K. M. Shakesheff, M. C. Davies, C. J. Roberts, S. J. B. Tendler. Surface plasmon resonance analysis of dynamic biological interactions with biomaterials. Biomaterials, 21, 1823-1835(2000).
[4] J. Homola, I. Koudela, S. S. Yee. Surface plasmon resonance sensors based on diffraction gratings and prism couplers: sensitivity comparison. Sens. Actuat. B: Chem., 54, 16-24(1999).
[5] I. Abdulhalim, M. Zourob, A. Lakhtakia. Surface plasmon resonance for biosensing: a mini-review. Electromagnetics, 28, 214-242(2008).
[6] S. K. Arya, A. Chaubey, B. D. Malhotra. Fundamentals and applications of biosensors. Proc. Ind. Nat. Acad. Sci., 72, 249-266(2006).
[7] J. H. T. Luong, K. B. Male, J. D. Glennon. Biosensor technology: technology push versus market pull. Biotechnol. Adv., 26, 492-500(2008).
[8] S. E. Swiontek, D. P. Pulsifer, A. Lakhtakia. Optical sensing of analytes in aqueous solutions with a multiple surface-plasmon-polariton-wave platform. Sci. Rep., 3, 1409(2013).
[9] P. Yeh, A. Yariv, C.-S. Hong. Electromagnetic propagation in periodic stratified media. I. General theory. J. Opt. Soc. Am., 67, 423-438(1977).
[10] P. Yeh, A. Yariv, A. Y. Cho. Optical surface waves in periodic layered media. Appl. Phys. Lett., 32, 104-105(1978).
[11] M. Shinn, W. M. Robertson. Surface plasmon-like sensor based on surface electromagnetic waves in a photonic band-gap material. Sens. Actuat. B, 105, 360-364(2005).
[12] V. N. Konopsky, E. V. Alieva. Photonic crystal surface waves for optical biosensors. Anal. Chem., 79, 4729-4735(2007).
[13] P. Rivolo, F. Michelotti, F. Frascella, G. Digregorio, P. Mandracci, L. Dominici, F. Giorgis, E. Descrovi. Real time secondary antibody detection by means of silicon-based multilayers sustaining Bloch surface waves. Sens. Actuat. B: Chem., 161, 1046-1052(2012).
[14] A. Lakhtakia, J. A. Polo. Dyakonov-Tamm wave at the planar interface of a chiral sculptured thin film and an isotropic dielectric material. J. Eur. Opt. Soc. Rapid Pub., 2, 07021(2007).
[15] D. P. Pulsifer, M. Faryad, A. Lakhtakia. Observation of the Dyakonov-Tamm wave. Phys. Rev. Lett., 111, 243902(2013).
[16] D. P. Pulsifer, M. Faryad, A. Lakhtakia, A. S. Hall, L. Liu. Experimental excitation of the Dyakonov–Tamm wave in the grating-coupled configuration. Opt. Lett., 39, 2125-2128(2014).
[17] A. Lakhtakia, M. Faryad. Theory of optical sensing with Dyakonov–Tamm waves. J. Nanophoton., 8, 083072(2014).
[18] A. Lakhtakia, R. Messier. Sculptured Thin Films: Nanoengineered Morphology and Optics(2005).
[19] T. G. Mackay, A. Lakhtakia. Modeling chiral sculptured thin films as platforms for surface-plasmonic-polaritonic optical sensing. IEEE Sens. J., 12, 273-280(2012).
[20] I. J. Hodgkinson, Q. h. Wu, J. Hazel. Empirical equations for the principal refractive indices and column angle of obliquely-deposited films of tantalum oxide, titanium oxide and zirconium oxide. Appl. Opt., 37, 2653-2659(1998).
[21] N. S. Kapany, J. J. Burke. Optical Waveguides(1972).