Abstract
The gravimeter based on atom interferometry has potential wide applications on building gravity networks and geophysics as well as gravity assisted navigation. Here, we demonstrate experimentally a portable atomic gravimeter operating in the noisy urban environment. Despite the influence of noisy external vibrations, our portable atomic gravimeter reaches a sensitivity as good as and a resolution of 1.1 μGal after 4000 s integration, being comparable to state-of-the-art atomic gravimeters. Our achievement paves the way for bringing the portable atomic gravimeter to field applications.The atomic gravimeter offers a new concept for both very sensitive and accurate absolute gravity measurement[1,2]. This technology can reach a best short-term sensitivity of a few micro-Galileo per square root of Hertz () that outperforms state-of-the-art classical corner cube sensors[3–16]. Nowadays, the development of portable atomic gravimeters overcomes many limitations of existing sensors and opens wide area of potential applications in geophysics, resource exploration, gravity field mapping, earthquake prediction as well as gravity-aided navigation[17–26]. More and more efforts have been made in the field applications of atomic gravimeters recently. Desruelle has deployed a commercial portable atomic gravimeter on Mount Etna for monitoring the gravity anomaly induced by volcanic activity[27]. Mounted on an inertial stabilized platform, an atomic gravimeter was applied to measure the gravity in the Atlantic as a marine gravimeter[28]. A vehicle atomic gravimeter is also operated for the gravity survey in hills[29].
Despite the high performance obtained in very quiet and well controlled laboratory conditions, such as cave laboratory[14], remote locations[1], and underground galleries[30,31], rare precise measurements with portable atomic gravimeters are reported in the noisy urban environment with high sensitivity and resolution, since the performance is largely limited by parasitic vibrations from the ground[11,12,15].
Rising to the challenge of operating a portable atomic gravimeter in the noisy urban environment, we develop a miniaturized atom sensor mounted on a mobile active vibration isolation stage. The portable atomic gravimeter is then transported to and operating in the urban environment for more than 10 days. With the external vibrations of the Raman mirror being suppressed simultaneously in three dimensions, the vibration noise is reduced by a factor up to 2000 from 0.01 Hz to 10 Hz in the vertical direction and by a factor up to 30 horizontally, allowing our portable atomic gravimeter to reach a sensitivity of () at night (daytime) and a resolution of after 4000 s integration.
Sign up for Chinese Optics Letters TOC. Get the latest issue of Chinese Optics Letters delivered right to you!Sign up now
The scheme of the portable atomic gravimeter operating in the urban environment is shown in Fig. 1(a). It includes a cold atom sensor head () that provides the gravity measurement and a mobile active vibration isolator () that suppresses the parasitic vibration from the ground. An integrated controller package () provides all of the lasers for manipulating cold atoms and performs data acquisition and processing. The total power consumption is less than 400 W.
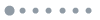
Figure 1.(a) Schematic diagram of the main science package, where the miniaturized atom sensor is mounted on the portable active vibration isolation platform. (b) Photo of the portable atomic gravimeter running in a noisy lab.
The miniaturized atom sensor consists of a titanium vacuum chamber, magnetic coils, optics for delivering laser beams, and collectors for fluorescence signals. The sensor is implemented in a compact two-layer magnetic shield, with residual magnetic field below 50 nT near the center. The atoms as test mass are loaded directly from the background vapor by the three-dimensional magneto-optical trap (3D MOT) in 120 ms and further cooled down to by optical molasses. Two optical-phase-locked Raman lasers are combined together and aligned carefully along the vertical direction with a retro-reflected configuration [Fig. 1(a)]. The initial state is prepared by a series of Raman pulses, during which atoms are selected with a temperature of 300 nK in the vertical direction and prepared in the magnetic insensitive state . Mach–Zehnder type matter wave interferometry is realized by shining a Raman-pulse sequence with time interval of and -pulse length . To prevent the incoherent photon scattering, the Raman lasers are detuned to the red of 700 MHz from the D2 transition lines. The Doppler effect during free fall is compensated by chirping the relative frequency of the Raman lasers at the rate of . After the interferometry, the atoms at are counted by collecting the fluorescence of each state, respectively. The interferometry fringe is obtained by scanning the chirping rate , and the gravity value is achieved via full-fringe fitting.
A homemade portable three-dimensional active vibration isolator underneath the atom sensor is applied to isolate the Raman retro-reflector from the ground vibration. The schematic overview of the active vibration isolator is shown in Fig. 2(a). It is based on a commercial passive vibration isolation platform (Minus-K Technology 50BM-4). Eight voice coil motors driven by homemade voltage controlled current sources (VCCSs) are added inside to implement the active feedback units. A precision three-axis seismometer (Guralp CMG-3ESP) mounted on the isolation platform is used to monitor the vibration noise of the retro-reflector. The residual vibration noises that go through an analog-digital converter are transferred to a programmable digital feedback filter with a sampling rate of 1 kHz. By passing through a digital-analog converter and the VCCS, the output signal from the filter is turned into current to drive the voice coil motors that implement the feedback force in three dimensions[32].
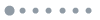
Figure 2.(a) Schematic overview of the three-dimensional active vibration isolator. (b) Vibration noise in the vertical direction. Red: the noise spectrum measured directly on the lab floor. Blue: the residual vibration noise on the passive isolator. Black: the residual vibration noise on the isolator with active feedback. (c) Long-term performance of the isolator. Inset: vibrational transfer function of the atom sensor.
In order to actively suppress the vibration in the vertical direction as well as possible, two filters are applied in the vertical feedback loop. One is a normal wide band low pass filter that suppresses most of the vibration noises and prevents the long-term drift. The other is a band-pass filter that further suppresses the noise from 0.1 Hz to 10 Hz, which affects our atomic gravimeter the most. In addition, the horizontal vibration signals are not only used to remove the vibrations in two horizontal directions, but are also taken into account in the vertical direction to prevent the crosstalk between the vertical vibrations and horizontal ones[32].
Eight voice coil motors are mounted at the symmetric positions in the active vibration isolator to provide the feedback forces evenly and to avoid any torques that may cause the flip.
The performance of the active vibration isolator is characterized by monitoring the residual vibrations with the seismometer. Figure 2(b) shows the equivalent vertical acceleration noise power spectrum in different situations. The red curve indicates the ground vibration noise at the assembly site, located in one of the labs at Shanghai Institute, University of Science and Technology of China. As the site suffers badly from heavy human activities and highway traffic nearby, the vibration noise below 10 Hz is much larger than , and the vibration eigenmode around 2.5 Hz[33] is as large as . Transformed to the noise of the gravity measurement, it is equivalent to the noise of higher than [34], which is impossible for the precision measurement at the level. It means that we are in a quite noisy urban environment compared to well established gravity measurement sites[13,35–37]. The blue curve indicates the residual vibration noise on the passive vibration isolation platform without feedback. The noise above 1 Hz is effectively suppressed. While, around the natural resonance frequency of the isolation platform, 0.5 Hz, the noise is slightly amplified. The black curve indicates the residual vibration noise of the Raman retro-reflector with the active feedback loop turned on. The residual vibration noise is reduced by a factor of 2000 from 0.01 Hz to 10 Hz, as the residual noise due to crosstalk between the horizontal and vertical directions is further suppressed by extra horizontal feedback channels. The vibration noise is also reduced horizontally by a factor of 30 from 0.01 Hz to 10 Hz (not shown here)[32]. The long-term performance of the three-dimensional active vibration isolator is presented in Fig. 2(c). We characterize it by monitoring the sensitivity suffered to the residual vibration noise for more than 10 days. It is maintained around with a standard deviation of . There are some outliers (mostly during the daytime) due to occasional human activities close to the setup. After that, the active vibration isolator recovers very quickly and works well for the whole period.
The laser system is realized using two diode lasers (DL-I and DL-II) and one tapered amplifier (TA) to provide all functions required for an atomic gravimeter. DL-I is locked on an atomic transition, serving as master Raman laser and repumper; the laser from DL-II is amplified by the TA and phase locked to DL-I, providing cooling, probe, and slave Raman laser and the “blow” laser that removes the atoms remaining in the undesired states. To simplify the laser setup, the acousto-optical modulators used as the optical switches for the functional lights are controlled by the same RF source with different transistor–transistor logic (TTL) switches. The versatile laser frequency tuning to perform laser cooling and interferometry is achieved by the optical phase lock loop with fast ramping offset frequency provided by a direct digital synthesizer. The lasers and optics are integrated in a sealed module to reach mechanical stability and compactness.
The electronics for the laser controller, time controller, as well as data acquisition modules are all integrated in three standard 3U 19 in. electronic boxes and mounted together with the optics module in a rack.
From the capture of cold atoms to the end of the interferometry, the full circle of the gravimeter takes about 300 ms and works at the repetition rate of 3 Hz. We perform the gravity measurements by Raman pulses interacting with the cold atoms during free falling. The interferometry fringe is obtained by scanning the chirping rate . We have where is the normalized population of atoms in the state, is the offset, is the amplitude of the interference fringes, is the effective wave vector of the Raman lasers, and is the interrogation time between Raman pulses. The gravity can be achieved via full-fringe fitting.
In order to reduce the -independent systematic errors, which include the quadratic Zeeman shift, the one photon light shift, and the RF phase shift[38], we flip the direction of by switching the sign of chirping rate for every 48 drops (16 s).
The interferometry fringes with the chirp up and chirp down during gravity measurements are shown in Fig. 3. The cosine function is applied to fit the interference pattern. At each fitting extreme maximum point for chirp up and chirp down, we obtain and , respectively, which mark the point with zero phase shift of the interferometer. By the least-squares fitting of the pattern, we obtain the fitting errors of and as and for a single interference fringe every 16 s.
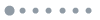
Figure 3.Interferometry fringe for . It is obtained by 48 drops in 16 s for chirp up and down, respectively. Each black dot is the probability of atoms in the state by the averaging of four drops. The error bar represents the statistical error. The purple and red lines are the fitting according to chirp up and down, respectively.
For obtaining the value, we derive and in Eq. (1) by counting the systematic errors as where represents the systematic phase shift, which is dependent (independent) on the direction of . We derive the expression of the from these two equations and obtain
The systematic phase shifts that are independent of the sign of are reduced, only the dependent term is left, which includes the effect of two-photon light shift, self-gravity, Coriolis forces, the wave-front aberrations, etc.[38].
From Eq. (3), we further obtain the noise level of each measurement: within 30 s integration time.
After the completion of the assembly and adjustment, the portable atomic gravimeter performs the continuous measurement of the local gravity over 245 h at the assembly site, from the 29th October to the 8th November 2019. As shown in Fig. 4, despite the influence of noisy external vibrations, the experimental results (black dots) agree well with Earth’s tides (red) predicted theoretically with an inelastic non-hydrostatic Earth model[39]. The fluctuation of the residue signal may come from the temperature variation in the lab.
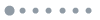
Figure 4.Top: the gravity acceleration measured by the portable atomic gravimeter between the 29th October and the 8th November 2019. The setup works continuously for more than 10 days in the noisy lab. The two breaks (from 130 h to 143 h and from 192 h to 201 h) are caused by the lasers out of lock. Bottom: the residue achieved from the corresponding gravity signal subtracted by Earth’s tides.
The Allan deviation of the residue signal is then calculated to characterize the sensitivity and long-term stability of our portable atomic gravimeter. As shown in Fig. 5, the sensitivity of the portable gravimeter follows for up to 1000 s during daytime and follows for up to 4000 s during nighttime. The level is obtained after about 60 s of measurement, and the Allan deviation continues to decrease down to () within an integration time of 4000 s (2000 s) during nighttime (daytime).
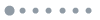
Figure 5.Allan deviation of the gravity signal corrected for Earth’s tides in the daytime (red) and at night (black). The slopes represent the corresponding averaging expected for white noise.
For nearby locations, the portable atomic gravimeter is mobile enough to be deployed and perform gravity measurements in the field with handling by only one or two people. Meanwhile, it can also fit inside a minivan and be robust enough to be transported in the long range, for example, we successfully accomplish a comparison mission after 1300-km-long transportation from Shanghai to Beijing[32].
In conclusion, we demonstrate that the portable atomic gravimeter operates well in the noisy urban environment with the help of a portable three-dimensional active vibration isolator. The portable atomic gravimeter reaches a sensitivity as good as and a resolution of within 4000 s integration. Moreover, the setup is robust enough to be deployed in the long range and to perform gravity measurements with handling by one or two people. The technique demonstrated here helps us to push the portable atomic gravimeter to field applications where gravity survey has to be performed in the noisy environment. Furthermore, with the flexibility of being mounted on a vehicle or a gyro-stabilized platform[28,29], the demonstration would be of interest for applications of the atom sensor using active vibration isolation to mobile gravity survey or inertial navigation.
References
[1] C. Freier, M. Hauth, V. Schkolnik, B. Leykauf, M. Schilling, H. Wziontek, H.-G. Scherneck, J. Müller, A. Peters. J. Phys.: Conf. Ser., 723, 012050(2016).
[2] S. Dickerson, J. M. Hogan, A. Sugarbaker, D. M. S. Johnson, M. A. Kasevich. Phys. Rev. Lett., 111, 083001(2013).
[3] S. Wang, Y. Zhao, W. Zhuang, T. Li, S. Wu, J. Feng, C. Li. Metrologia, 55, 360(2018).
[4] T. Mazzoni, X. Zhang, R. P. D. Aguila, L. Salvi, N. Poli, G. M. Tino. Phys. Rev. A, 92, 0536191(2015).
[5] X. Zhang, J. Zhong, B. Tang, X. Chen, L. Zhu, P. Huang, J. Wang, M. Zhan. Appl. Opt., 57, 6545(2018).
[6] Q. Bodart, S. Merlet, N. Malossi, F. P. D. Santos, P. Bouyer, A. Landragin. Appl. Phys. Lett., 96, 134101(2010).
[7] R. Charriere, M. Cadoret, N. Zahzam, Y. Bidel, A. Bresson. Phys. Rev. A, 85, 013639(2012).
[8] N. Poli, F. Y. Wang, M. Tarallo, A. Alberti, M. Prevedelli, G. M. Tino. Phys. Rev. Lett., 106, 038501(2011).
[9] L. Zhou, Z. Xiong, W. Yang, B. Tang, W. Peng, Y. Wang, P. Xu, J. Wang, M. Zhan. Chin. Phys. Lett., 28, 013701(2011).
[10] P. A. Altin, M. Johnsson, V. Negnevitsky, G. Dennis, R. P. Anderson, J. E. Debs, S. S. Szigeti, K. S. Hardman, S. Bennetts, G. Mcdonald. New J. Phys., 15, 023009(2013).
[11] Y. Bidel, O. Carraz, R. Charriere, M. Cadoret, N. Zahzam, A. Bresson. Appl. Phys. Lett., 102, 144107(2013).
[12] L. Zhou, Z. Xiong, W. Yang, B. Tang, W. Peng, K. Hao, R. Li, M. Liu, J. Wang, M. Zhan. Gen. Relativ. Gravitation, 43, 1931(2011).
[13] M. Hauth, C. Freier, V. Schkolnik, A. Senger, M. Schmidt, A. Peters. Appl. Phys. B, 113, 49(2013).
[14] Z. K. Hu, B. L. Sun, X. C. Duan, M. K. Zhou, L. L. Chen, S. Zhan, Q. Z. Zhang, J. Luo. Phys. Rev. A, 88, 043610(2013).
[15] B. Wu, Z. Wang, B. Cheng, Q. Wang, A. Xu, Q. Lin. Metrologia, 51, 452(2014).
[16] Z. J. Fu, Q. Y. Wang, Z. Y. Wang, B. Wu, B. Cheng, Q. Lin. Chin. Opt. Lett., 17, 080602(2019).
[17] G. Dagostino, S. Desogus, A. Germak, C. Origlia, D. Quagliotti, G. Berrino, G. Corrado, V. Derrico, G. Ricciardi. Ann. Geophys., 51, 39(2008).
[18] F. Greco, G. Currenti, G. Dagostino, A. Germak, R. Napoli, A. Pistorio, C. D. Negro. Bull. Volcanol., 74, 1745(2012).
[19] U. Dogan, S. Ergintav, G. Arslan, D. O. Demir, B. Karaboce, E. Bilgic, E. Sadikoglu, A. Direnc. Acta Geod. Geophys., 48, 377(2013).
[20] J. Montagner, K. Juhel, M. Barsuglia, J. Ampuero, E. Chassandemottin, J. Harms, B. F. Whiting, P. Bernard, E. Clevede, P. Lognonne. Nat. Commun., 7, 13349(2016).
[21] T. Jacob, J. Chery, R. Bayer, N. L. Moigne, J. P. Boy, P. Vernant, F. Boudin. Geophys. J. Int., 177, 347(2009).
[22] J. T. Reager, B. F. Thomas, J. S. Famiglietti. Nat. Geosci., 7, 588(2014).
[23] M. N. Nabighian, M. E. Ander, V. J. S. Grauch, R. O. Hansen, T. R. Lafehr, Y. Li, W. C. Pearson, J. W. Peirce, J. D. Phillips, M. E. Ruder. Geophysics, 70, 63ND(2005).
[24] I. Velicogna, J. Wahr. Science, 311, 1754(2006).
[25] N. Yu, J. M. Kohel, J. R. Kellogg, L. Maleki. Appl. Phys. B, 84, 647(2006).
[26] A. B. Chatfield(1997).
[27] B. Desruelle. LASER 2019: quantum sensor set for trip to Mount Etna(2019).
[28] Y. Bidel, N. Zahzam, C. Blanchard, A. Bonnin, M. Cadoret, A. Bresson, D. Rouxel, M. F. Lequentreclalancette. Nat. Commun., 9, 627(2018).
[29] X. Wu, Z. Pagel, B. S. Malek, T. H. Nguyen, F. Zi, D. S. Scheirer, H. Muller. Sci. Adv., 5, 9(2019).
[30] P. Gillot, O. Francis, A. Landragin, F. P. D. Santos, S. Merlet. Metrologia, 51, L15(2014).
[31] T. Farah, C. Guerlin, A. Landragin, P. Bouyer, S. Gaffet, F. P. D. Santos, S. Merlet. Gyroscopy Navig., 5, 266(2014).
[32] B. Chen, J. B. Long, H. T. Xie, L. K. Chen, S. Chen. Acta Phys. Sin., 68, 183301(2019).
[33] C. Freier. Measurement of local gravity using atom interferometry. University of Humboldt(2010).
[34] P. Cheinet, B. Canuel, F. P. D. Santos, A. Gauguet, F. Yverleduc, A. Landragin. IEEE Trans. Instrum. Meas, 57, 1141(2008).
[35] J. L. L. Gouet, T. E. Mehlstaubler, J. Kim, S. Merlet, A. Clairon, A. Landragin, F. P. D. Santos. Appl. Phys. B, 92, 133(2008).
[36] S. Merlet, J. L. L. Gouet, Q. Bodart, A. Clairon, A. Landragin, F. P. D. Santos, P. Rouchon. Metrologia, 46, 87(2009).
[37] M. Zhou, Z. Hu, X. Duan, B. Sun, L. Chen, Q. Zhang, J. Luo. Phys. Rev. A, 86, 043630(2012).
[38] A. Louchetchauvet, T. Farah, Q. Bodart, A. Clairon, A. Landragin, S. Merlet, F. P. D. Santos. New J. Phys., 13, 065025(2011).
[39] V. Dehant, P. Defraigne, J. M. Wahr. J. Geophys. Res.: Solid Earth, 104, 1035(1999).