
- Photonics Research
- Vol. 9, Issue 10, 2088 (2021)
Abstract
1. INTRODUCTION
Controlling the propagation of electromagnetic (EM) waves in a predesigned way is highly expected due to curiosity in fundamental physics and increasing technological demands in imaging and telecommunications. The bulky dielectric refractive lenses and parabolic mirrors are conventional lenses for focusing and imaging. The resolution of conventional lenses is confined to
To solve the intrinsic problem of superlenses and hyperlenses, the superoscillation lens (SOL) has been proposed. Based on the mechanism of superoscillation [14–17], many scalar SOLs [18–20] and vector SOLs [21–23] have been designed to accomplish far-field superresolution without evanescent waves. However, these SOLs’ working bandwidth is restricted to just one single frequency [24] or some discrete frequency points [25]. Even worse, when the resolution increases, the sidelobe of the focusing spot in the far field will dramatically rise [26]. To overcome the drawbacks of SOL, supercritical lenses (SCLs) [27,28] and photon sieves [29] were proposed. Ultralong working distance and subdiffraction focusing (
Moreover, gradient refractive index (GRIN) lenses have presented excellent performance in focusing [42–46], imaging [47–49], magnifying the far field [50,51], and beam forming [52,53]. The Maxwell fish-eye (MFE) lens [54] has been validated to generate an achromatic image with a large NA. Consequently, a new semicircular gradient index lens (sGRIN) with a modified RI distribution is proposed. Broadband (7–13 GHz) highly efficient (above 89%) achromatic subdiffraction focusing at the focusing line with full width at half-maximum (FWHM) around
Sign up for Photonics Research TOC. Get the latest issue of Photonics Research delivered right to you!Sign up now
2. THEORETICAL DESIGN of sGRIN
Let us start from the RI profile of the classical half-MFE lens as follows:
Figure 1.Focusing performance of sGRIN. (a) RI profile of sGRIN; (b) map of electric near-field intensity profile at the focusing line in the frequency range of 4 to 20 GHz; (c) calculated electric near-field intensity distribution; (d) related FWHM; (e) focusing efficiency of proposed sGRIN.
For obtaining the lowest reflection at the lower side of the lens/air interface and widest operating frequency band, through numerous numerical simulations via the exhaustive method for the
According to the reciprocity principle [56], the process of transforming EM wave propagation with low spatial frequency in air into EM wave propagation with high spatial frequency in sGRIN to generate subdiffraction focusing [Fig. 1(c)] can be reversed. This is confirmed by applying the two-point sources technique, which has been widely adopted to determine the resolution of an optical system. Two-point sources are located at the vertex of the sGRIN separated by
Figure 2.Principle of diffraction-limited far-field radiation of sGRIN. (a) sGRIN with RI distribution of
3. STRUCTURAL DESIGN AND SIMULATED RESULTS
Owing to the fact that the RI distribution of the designed lens originating from the classical half-MFE (HMFE) lens is nonmagnetic, inhomogeneous, and isotropic, all dielectric nonresonant metamaterials could be applied. For this specific RI profile varying from
Figure 3.Design and simulated results of sGRIN. (a) RI profile of sGRIN (dotted red line) and the discrete (solid blue line) one. The inset depicts three kinds of unit cells adopted based on the three regions in sGRIN. (b) Schematic of sGRIN; (c) map of simulated near-field intensity profile at the focusing line from 4 to 20 GHz; comparison between simulated (d) FWHM and (e) focusing efficiency of sGRIN with a discrete RI distribution (red solid line) and that of the original sGRIN with a continuous RI distribution (blue dashed line); (f) map of simulated normalized far-field intensity profile; (g) related well depth; distance between two feeding sources.
To demonstrate the effectiveness of our designed unit cells, the subdiffraction focusing and diffraction-limited far-field radiation pattern of the designed lens with a discrete RI distribution are simulated. For the subdiffraction focusing performance, the map of the near-field electric intensity profile at the focusing line with respect to the frequency range from 4 to 20 GHz is given in Fig. 3(c). The focusing band becomes narrower and narrower with the frequency increasing. In line with the FWHM tendency in Fig. 3(d), it is found that the FWHM of designed structure varies from
The far-field radiation pattern of the designed lens is investigated by analyzing its well depth. Figure 3(f) presents the map of the normalized far-field radiation intensity profile fed by two sources with a distance of
The designed discrete subdiffraction focusing lens is manufactured by computer numerical control (CNC) machining technology and 3D printing technology.
4. EXPERIMENTAL RESULTS and DISCUSSION
Figure 4(a) is the photograph of the fabricated sample. Both the near-field electric distribution and the far-field radiation pattern are measured to validate the function of subdiffraction focusing and diffraction-limited far-field radiation. The scanning system proposed in Ref. [57] is applied to test the near-field electric distribution. An incident plane wave passing through the sample converges at the vertex of the lens, and a trenchant focus is yielded. Figures 4(b) and 4(c) describe the map of electric intensity distribution at the focusing line and related near-field electric intensity profile with plane wave illuminating the lens from 7 to 13 GHz. Besides a tiny focal spot emerging at the peak of the sample, a notable cylindrical wavefront is also generated inside the lens. The upper inset drawings in Fig. 4(c) present the FWHM along the
Figure 4.Experimental results of subdiffraction focusing and diffraction-limited far-field radiation. (a) Photograph of the fabricated sample; (b) experimental map of near-field intensity profile at the focusing line from 7 to 13 GHz; (c) measured electric near-field intensity distribution and the corresponding FWHM of the combined lenses; comparison between the experimental (d) FWHM and (e) focusing efficiency of sGRIN (red solid line) with calculated results of sGRIN with a continuous RI distribution (dashed blue line); (f) schematic of the lens antenna; (g) map of measured far-field radiation intensity profiles fed by two sources with a subwavelength distance
The sample is tested in a full anechoic chamber to get the diffraction-limited far-field radiation pattern. The sketch map of the lens antenna fed by two sources is depicted in Fig. 4(f). The experimental map of the normalized far-field radiation intensity profile fed by two sources is depicted in Fig. 4(g). Two main beams are clearly observed, and the related well depths are demonstrated in Fig. 4(h). The well depths are all below
Figure 5 displays the comparison of half of the light cone angle, operational bandwidth, and focusing efficiency among SIL, negative refractive lens (NRI), SOL, hyperlens (HPL), and our designed sGRIN, respectively. It is clear that our designed lens demonstrates the best overall performance in the field of subdiffraction lenses. Generally speaking, an achromatic subdiffraction sGRIN is realized with the proposed design method. It is shown that the designed discrete lens’ performance in both near field and far field agrees well with the theoretical prediction, which means that the proposed sGRIN has great potential to be used in subdiffraction imaging systems in the future.
Figure 5.Comparison of half of the light cone angle, operational bandwidth, and focusing efficiency among SIL, NRL, SOL, HPL, and our designed sGRIN.
5. DISCUSSIONS AND OUTLOOK
In summary, a semicircular all-dielectric GRIN lens with a slightly modified RI distribution of classical half-MFE lens is designed to achieve achromatic subdiffraction focusing with the FWHM around
Acknowledgment
Acknowledgment. We thank Tianyu Dong for helpful discussions and Wei Li for help with the lens antenna setup.
References
[1] E. Abbe. Resolution of microscopes. Arch. Mikrosk. Anat, 9, 413-468(1873).
[2] J. B. Pendry. Negative refraction makes a perfect lens. Phys. Rev. Lett., 85, 3966-3969(2000).
[3] N. Fang, H. Lee, C. Sun, X. Zhang. Negative refraction makes a perfect lens. Science, 308, 534-537(2005).
[4] T. Taubner, D. Korobkin, Y. Urzhumov, G. Shvets, R. Hillenbrand. Near-field microscopy through a SiC superlens. Science, 313, 1595(2006).
[5] E. Cubukcu, K. Aydin, E. Ozbay, S. Foteinopoulou, C. M. Soukoulis. Subwavelength resolution in a two-dimensional photonic-crystal-based superlens. Phys. Rev. Lett., 91, 207401(2003).
[6] K. Aydin, I. Bulu, E. Ozbay. Subwavelength resolution with a negative-index metamaterial superlens. Appl. Phys. Lett., 90, 254102(2007).
[7] W. Cai, D. A. Genov, V. M. Shalaev. Superlens based on metal-dielectric composites. Phys. Rev. B, 72, 193101(2005).
[8] V. A. Podolskiy, E. E. Narimanov. Near-sighted superlens. Opt. Lett, 30, 75-77(2005).
[9] A. Salandrino, N. Engheta. Far-field subdiffraction optical microscopy using metamaterial crystals: theory and simulations. Phys. Rev. B, 74, 075103(2006).
[10] Z. Jacob, L. V. Alekseyev, E. Narmanov. Optical hyperlenses: far-field imaging beyond the diffraction limit. Opt. Express, 14, 8247-8256(2006).
[11] Z. W. Liu, H. Lee, Y. Xiong, C. Sun, X. Zhang. Far-field optical hyperlens magnifying sub-diffraction-limited objects. Science, 315, 1686(2007).
[12] J. B. Sun, M. I. Shalaev, N. M. Litchinitser. Experimental demonstration of a non-resonant hyperlens in the visible spectral range. Nat. Commun, 6, 7201(2015).
[13] J. Rho, Z. Ye, Y. Xiong, X. Yin, Z. W. Liu, H. Choi, G. Bartal, X. Zhang. Spherical hyperlens for two-dimensional sub-diffractional imaging at visible frequencies. Nat. Commun., 1, 143(2010).
[14] D. Z. Albert, L. Vaidman, Y. Aharonov. How the result of a measurement of a component of the spin of a spin-1/2 particle can turn out to be 100. Phys. Rev. Lett., 60, 1351-1354(1988).
[15] M. V. Berry. Evanescent and real waves in quantum billiards and Gaussian beams. J. Phys. A, 27, L391-L398(1994).
[16] M. V. Berry, S. Popescu. Evolution of quantum superoscillations and optical super resolution without evanescent waves. J. Phys. A, 39, 6965-6977(2006).
[17] K. Huang, H. P. Ye, J. H. Teng, S. P. Yeo, B. Luk’yanchuk, C.-W. Qiu. Optimization-free superoscillatory lens using phase and amplitude masks. Laser Photon. Rev., 8, 152-157(2014).
[18] E. T. F. Rogers, J. Lindberg, T. Roy, S. Savo, J. E. Chad, M. R. Dennis, N. I. Zheludev. A super-oscillatory lens optical microscope for subwavelength imaging. Nat. Mater., 11, 432-435(2012).
[19] K. S. Rogers, E. T. F. Rogers, N. I. Zheludev, G. Yuan. Far-field superoscillatory metamaterial superlens. Phys. Rev. Appl., 11, 024073(2019).
[20] Q. Zhang, F. Dong, H. Li, Z. Wang, G. Liang, Z. Zhang, Z. Wen, Z. Shang, G. Chen, L. Dai, W. Chu. High-numerical-aperture dielectric metalens for super-resolution focusing of oblique incident light. Adv. Opt. Mater., 8, 1901885(2020).
[21] R. Zuo, W. Liu, H. Cheng, S. Chen, J. Tian. Breaking the diffraction limit with radially polarized light based on dielectric metalenses. Adv. Opt. Mater., 6, 1800795(2018).
[22] L. Chen, J. Liu, X. Zhang, D. Tang. Achromatic super-oscillatory metasurface through optimized multiwavelength functions for sub-diffraction focusing. Opt. Lett., 45, 5772-5775(2020).
[23] Z. Wu, F. Dong, S. Zhang, S. Yan, G. Liang, Z. Zhang, Z. Wen, G. Chen, L. Dai, W. Chu. Broadband dielectric metalens for polarization manipulating and superoscillation focusing of visible light. ACS Photon., 7, 180-189(2020).
[24] D. Tang, C. Wang, Z. Zhao, Y. Wang, M. Pu, X. Li, P. Gao, X. Luo. Ultrabroadband superoscillatory lens composed by plasmonic metasurfaces for sub-diffraction light focusing. Laser Photon. Rev., 9, 713-719(2015).
[25] D. Tang, L. Chen, J. Liu. Visible achromatic super-oscillatory metasurfaces for sub-diffraction focusing. Opt. Express, 27, 12308-12316(2019).
[26] E. T. Rogers, N. I. Zheludev. Optical super-oscillations: sub-wavelength light focusing and super-resolution imaging. J. Opt., 15, 094008(2013).
[27] F. Qin, K. Huang, J. Wu, J. Teng, C.-W. Qiu, M. Hong. A supercritical lens optical label-free microscopy: sub-diffraction resolution and ultra-long working distance. Adv. Mater., 29, 1602721(2017).
[28] C. L. Hao, Z. Q. Nie, H. P. Ye, H. Li, Y. Luo, R. Feng, X. Yu, F. Wen, Y. Zhang, C. Y. Yu, J. H. Teng, B. Luk’yanchuk, C.-W. Qiu. Three-dimensional supercritical resolved light-induced magnetic holography. Sci. Adv., 3, e1701398(2017).
[29] K. Huang, H. Liu, F. J. Garcia-Vidal, M. H. Hong, B. Luk’yanchuk, J. H. Teng, C.-W. Qiu. Ultrahigh-capacity non-periodic photon sieves operating in visible light. Nat. Commun., 6, 7059(2015).
[30] X. Lu, Y. Guo, M. Pu, Y. Zhang, Z. Li, X. Ma, X. Luo. Broadband achromatic metasurfaces for sub-diffraction focusing in the visible. Opt. Express, 29, 5947-5958(2021).
[31] Y. J. Bao, J. C. Ni, C.-W. Qiu. A minimalist single-layer metasurface for arbitrary and full control of vector vortex beams. Adv. Mater., 32, 1905659(2020).
[32] S. Chen, Z. Li, W. Liu, H. Cheng, J. Tian. From single-dimensional to multidimensional manipulation of optical waves with metasurfaces. Adv. Mater., 31, 1802458(2019).
[33] S. Chen, W. W. Liu, Z. C. Li, H. Cheng, J. G. Tian. Metasurface-empowered optical multiplexing and multifunction. Adv. Mater., 32, 2070022(2020).
[34] S. Wang, P. C. Wu, V. C. Su, Y. C. Lai, M. K. Chen, H. Y. Kuo, B. H. Chen, Y. H. Chen, T. T. Huang, J. H. Wang. A broadband achromatic metalens in the visible. Nat. Nanotechnol, 13, 227-232(2018).
[35] A. Ndao, L. Y. Hsu, J. Ha, J.-H. Park, C. Chang-Hasnain, B. Kante. Octave bandwidth photonic fishnet-achromatic-metalens. Nat. Commun., 11, 3205(2020).
[36] X. Luo, D. Tsai, M. Gu, M. Hong. Extraordinary optical fields in nanostructures: from sub-diffraction-limited optics to sensing and energy conversion. Chem. Soc. Rev., 48, 2494-2548(2019).
[37] S. Shrestha, A. C. Overvig, M. Lu, A. Stein, N. Yu. Broadband achromatic dielectric metalenses. Light Sci. Appl., 7, 85(2018).
[38] S. Wang, P. C. Wu, V. C. Su, Y. C. Lai, C. H. Chu, J. W. Chen, S. H. Lu, J. Chen, B. Xu, C. H. Kuan. Broadband achromatic optical metasurface devices. Nat. Commun., 8, 187(2017).
[39] S. M. Mansfield, G. S. Kino. Solid immersion microscope. Appl. Phys. Lett., 57, 2615-2616(1990).
[40] A. Bogucki, L. Zinkiewiz, M. Grzeszczyk, W. Pacuski, K. Nogajewski, T. Kazimierczuk, A. Rodek, J. Suffczynski, K. Watanabe, T. Taniguchi, P. Wasylczyk, M. Potemski, P. Kossacki. Ultra-long-working-distance spectroscopy of single nanostructures with aspherical solid immersion microlenses. Light Sci. Appl., 9, 48(2020).
[41] M.-S. Kim, T. Scharf, M. T. Haq, W. Nakagawa, H. P. Herzig. Subwavelength-size solid immersion lens. Opt. Lett., 36, 3930-3932(2011).
[42] J. Chen, X. Yuan, M. Chen, X. Cheng, A. Zhang, G. Peng, W. L. Song, D. Fang. Ultrabroadband three-dimensional printed radial perfectly symmetric gradient honeycomb all-dielectric dual-directional lightweight planar Luneburg lens. ACS Appl. Mater. Interfaces, 10, 38404-38409(2018).
[43] J. Chen, Y. Lin, G. Peng, Y. Huang, A. Zhang, W. L. Song, M. Chen, Z. Liu, D. Fang. An all-dielectric 3D Luneburg lens constructed by common-vertex coaxial circular cones. J. Phys. D, 53, 015110(2020).
[45] J. Chen, H. Chu, Y. Huang, Y. Lai, M. Chen, Z. Liu, D. Fang. Ultrabroadband compact lens antenna with high performance based on a transmission gradient index medium. J. Phys. D, 54, 175101(2020).
[46] J. Chen, H. Chu, Y. Lai, Z. Liu, H. Chen, M. Chen, D. Fang. Conformally mapped Mikaelian lens for broadband achromatic high resolution focusing. Laser Photon. Rev., 15, 2000564(2021).
[47] C. Guo, T. Urner, S. Jia. 3D light-field endoscopic imaging using a GRIN lens array. Appl. Phys. Lett., 116, 101105(2020).
[48] M. G. Scopelliti, M. Chamanzar. Ultrasonically sculpted virtual relay lens for
[49] J. Nagar, S. D. Campbell, D. H. Werner. Apochromatic singlets enabled by metasurface-augmented GRIN lenses. Optica, 5, 99-102(2018).
[50] W. Jiang, C.-W. Qiu, T. Han, Q. Cheng, H. Ma, S. Zhang, T. Cui. Broadband all-dielectric magnifying lens for far-field high-resolution imaging. Adv. Mater., 25, 6963-6968(2013).
[51] W. Jiang, S. Ge, T. Han, S. Zhang, M. Mehmood, C.-W. Qiu, T. Cui. Shaping 3D path of electromagnetic waves using gradient-refractive-index metamaterials. Adv. Sci., 3, 1600022(2016).
[52] N. Zhang, W. X. Jiang, H. F. Ma, W. X. Tang, T. J. Cui. Compact high-performance lens antenna based on impedance-matching gradient-index metamaterials. IEEE. Trans. Antennas. Propag., 67, 1323-1328(2018).
[53] A. Forbes. Common elements for uncommon light: vector beams with GRIN lenses. Light Sci. Appl., 8, 111(2019).
[54] O. Bitton, R. Bruch, U. Leonhardt. Two-dimensional Maxwell fisheye for integrated optics. Phys. Rev. Appl., 10, 044059(2018).
[55] A. Arbabi, Y. Horie, A. J. Ball, M. Bagheri, A. Faraon. Subwavelength-thick lenses with high numerical apertures and large efficiency based on high-contrast transmitarrays. Nat. Commun., 6, 7069(2013).
[56] R. J. Potton. Reciprocity in optics. Rep. Prog. Phys., 67, 717-754(2004).
[57] D. Schurig, J. J. Mock, B. J. Justice, S. A. Cummer, J. B. Pendry, A. F. Starr, D. R. Smith. Metamaterial electromagnetic cloak at microwave frequencies. Science, 314, 977-980(2006).
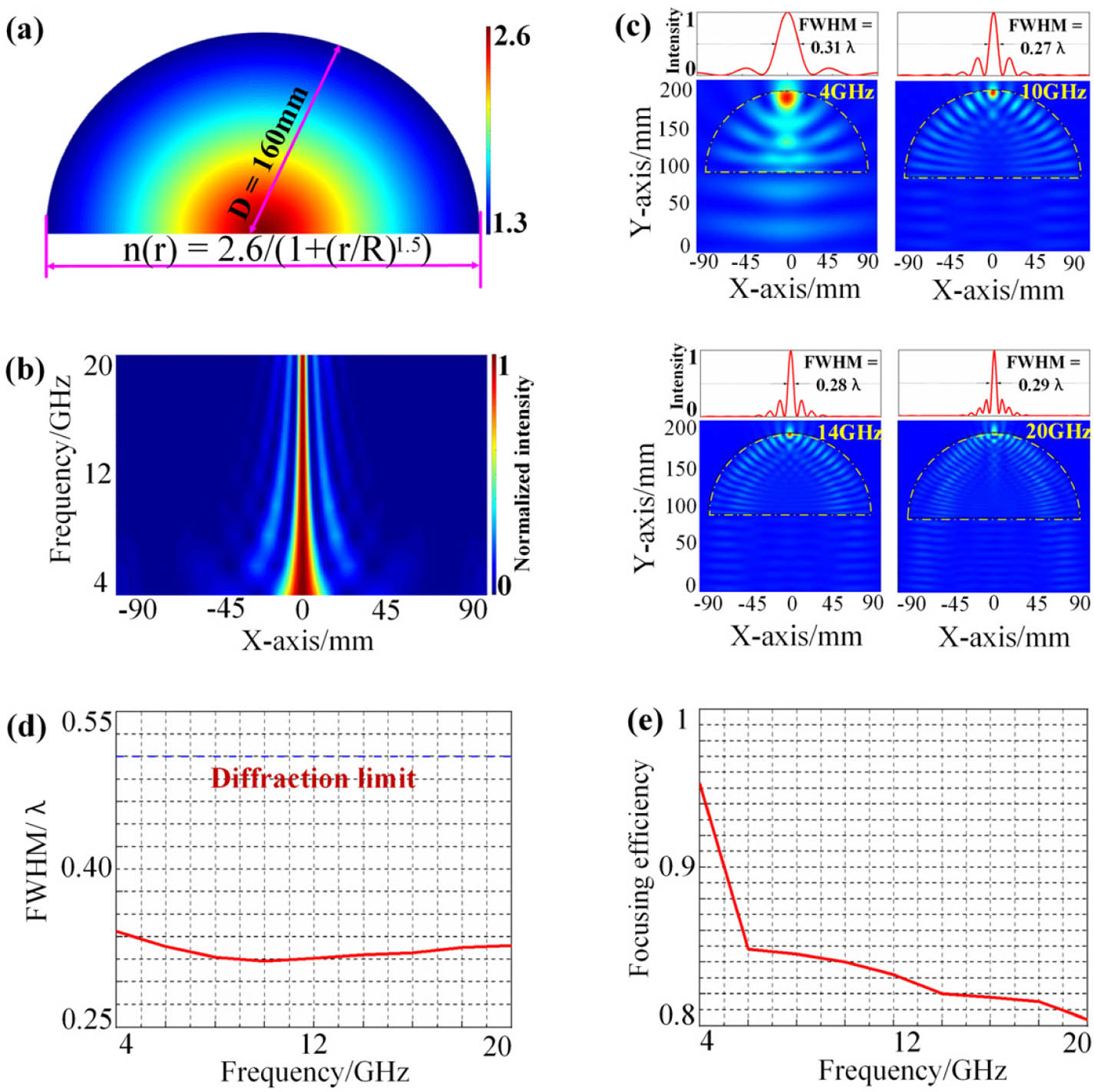
Set citation alerts for the article
Please enter your email address