
- Chinese Optics Letters
- Vol. 19, Issue 10, 103001 (2021)
Abstract
1. Introduction
The strong coupling between the crystal lattice and excitons would lead to the generation of self-trapped excitons (STEs)[
Recent studies reveal that two-dimensional (2D) perovskites show strong broadband luminescence, which is attributed to STE emission[
Here, we report on the identification of STE in 2D perovskite crystals by Raman spectroscopy. By measuring the Raman spectra of the
Sign up for Chinese Optics Letters TOC. Get the latest issue of Chinese Optics Letters delivered right to you!Sign up now
2. Crystal Preparation
Figure 1.(a) Schematic of
3. Self-Trapped Exciton Emission
Figure 2 displays the photoluminescence (PL) spectra of as-synthesized crystals at 80 K under different laser excitation. Under a 2.62 eV laser excitation, which is larger than the excitonic energy of
Figure 2.PL spectra of a
We have further carried out temperature-dependent PL studies. Figures 3 and 4 show the temperature-dependent PL spectra excited by the 2.33 eV and 1.96 eV lasers, respectively. We intentionally plot them at the same intensity scale for comparison. With the increase of temperature, the intensity of STE emission gradually decreases under the 2.33 eV laser excitation [Fig. 3(a)], while no emission peak is present for all of temperatures we investigated under a 1.96 eV excitation [Fig. 3(b)]. This further confirms that STE must be formed under excited states and independent of the surrounding temperature.
Figure 3.PL spectra of a
Figure 4.Raman spectra of a
4. Raman Spectroscopy
We have measured the Raman spectra of
In addition, compared with the spectrum under non-excited states,
In order to investigate the origin of
Figure 5.Temperature-dependent Raman spectra of a
The evolution of the Raman peak position with temperature under excited states and non-excited states is also extracted from Fig. 5 and plotted in Fig. 6. All Raman peaks exhibit a red shift as the temperature increases. Nevertheless,
Figure 6.Raman peak versus temperature extracted from Fig.
We have also measured the Raman spectra of
Figure 7.Raman spectra of
5. Conclusions
In summary, we have investigated the Raman spectra of
References
[1] R. T. Williams, K. S. Song. The self-trapped exciton. J. Phys. Chem. Solids, 51, 679(1990).
[2] H. Nishimura, T. Yamaoka, K.-I. Mizuno, M. Iemura, A. Matsui. Luminescence of free and self-trapped excitons in α-and β-perylene crystals. J. Phys. Soc. Jpn., 53, 3999(1984).
[3] X. Wu, M. T. Trinh, D. Niesner, H. Zhu, Z. Norman, J. S. Owen, O. Yaffe, B. J. Kudisch, X.-Y. Zhu. Trap states in lead iodide perovskites. J. Am. Chem. Soc., 137, 2089(2015).
[4] S. Kahmann, E. K. Tekelenburg, H. Duim, M. E. Kamminga, M. A. Loi. Extrinsic nature of the broad photoluminescence in lead iodide-based Ruddlesden–Popper perovskites. Nat. Commun., 11, 2344(2020).
[5] M. D. Smith, H. I. Karunadasa. White-light emission from layered halide perovskites. Acc. Chem. Res., 51, 619(2018).
[6] S. Li, J. Luo, J. Liu, J. Tang. Self-trapped excitons in all-inorganic halide perovskites: fundamentals, status, and potential applications. J. Phys. Chem. Lett., 10, 1999(2019).
[7] J. Li, H. Wang, D. Li. Self-trapped excitons in two-dimensional perovskites. Front. Optoelectron., 13, 225(2020).
[8] Q. Zhang, Y. Ji, Z. Chen, D. Vella, X. Wang, Q. H. Xu, Y. Li, G. Eda. Controlled aqueous synthesis of 2D hybrid perovskites with bright room-temperature long-lived luminescence. J. Phys. Chem. Lett., 10, 2869(2019).
[9] M. Cong, B. Yang, F. Hong, T. Zheng, Y. Sang, J. Guo, S. Yang, K. Han. Self-trapped exciton engineering for white-light emission in colloidal lead-free double perovskite nanocrystals. Sci. Bull., 65, 1078(2020).
[10] J. Luo, X. Wang, S. Li, J. Liu, Y. Guo, G. Niu, L. Yao, Y. Fu, L. Gao, Q. Dong, C. Zhao, M. Leng, F. Ma, W. Liang, L. Wang, S. Jin, J. Han, L. Zhang, J. Etheridge, J. Wang, Y. Yan, E. H. Sargent, J. Tang. Efficient and stable emission of warm-white light from lead-free halide double perovskites. Nature, 563, 541(2018).
[11] L. Mao, Y. Wu, C. C. Stoumpos, M. R. Wasielewski, M. G. Kanatzidis. White-light emission and structural distortion in new corrugated two-dimensional lead bromide perovskites. J. Am. Chem. Soc., 139, 5210(2017).
[12] E. R. Dohner, A. Jaffe, L. R. Bradshaw, H. I. Karunadasa. Intrinsic white-light emission from layered hybrid perovskites. J. Am. Chem. Soc., 136, 13154(2014).
[13] M. D. Smith, B. A. Connor, H. I. Karunadasa. Tuning the luminescence of layered halide perovskites. Chem. Rev., 119, 3104(2019).
[14] S. Zhang, Y. Zhong, F. Yang, Q. Cao, W. Du, J. Shi, X. Liu. Cavity engineering of two-dimensional perovskites and inherent light-matter interaction. Photon. Res., 8, A72(2020).
[15] T. Hu, M. D. Smith, E. R. Dohner, M.-J. Sher, X. Wu, M. T. Trinh, A. Fisher, J. Corbett, X.-Y. Zhu, H. I. Karunadasa, A. M. Lindenberg. Mechanism for broadband white-light emission from two-dimensional (110) hybrid perovskites. J. Phys. Chem. Lett., 7, 2258(2016).
[16] A. Yangui, D. Garrot, J. S. Lauret, A. Lusson, G. Bouchez, E. Deleporte, S. Pillet, E. E. Bendeif, M. Castro, S. Triki, Y. Abid, K. Boukheddaden. Optical investigation of broadband white-light emission in self-assembled organic-inorganic perovskite (C6H11NH3)2PbBr4. J. Phys. Chem. C, 119, 23638(2015).
[17] J. Li, J. Wang, J. Ma, H. Shen, L. Li, X. Duan, D. Li. Self-trapped state enabled filterless narrowband photodetections in 2D layered perovskite single crystals. Nat. Commun., 10, 806(2019).
[18] J. Wang, J. Li, Q. Tan, L. Li, J. Zhang, J. Zang, P. Tan, J. Zhang, D. Li. Controllable synthesis of two-dimensional Ruddlesden–Popper-type perovskite heterostructures. J. Phys. Chem. Lett., 8, 6211(2017).
[19] Y. Q. Zhao, Q. R. Ma, B. Liu, Z. L. Yu, J. Yang, M. Q. Cai. Layer-dependent transport and optoelectronic property in two-dimensional perovskite: (PEA)2PbI4. Nanoscale, 10, 8677(2018).
[20] Y.-T. Li, L. Ding, J.-Z. Li, J. Kang, D.-H. Li, L. Ren, Z.-Y. Ju, M.-X. Sun, J.-Q. Ma, Y. Tian, G.-Y. Gou, D. Xie, H. Tian, Y. Yang, L.-W. Wang, L.-M. Peng, T.-L. Ren. Light-enhanced ion migration in two-dimensional perovskite single crystals revealed in carbon nanotubes/two-dimensional perovskite heterostructure and its photomemory application. ACS Cent. Sci., 5, 1857(2019).
[21] Y. Liu, H. Ye, Y. Zhang, K. Zhao, Z. Yang, Y. Yuan, H. Wu, G. Zhao, Z. Yang, J. Tang, Z. Xu, S. Liu. Surface-tension-controlled crystallization for high-quality 2D perovskite single crystals for ultrahigh photodetection. Matter, 1, 465(2019).
[22] J. Li, J. Wang, Y. Zhang, H. Wang, G. Lin, X. Xiong, W. Zhou, H. Luo, D. Li. Fabrication of single phase 2D homologous perovskite microplates by mechanical exfoliation. 2D Mater., 5, 021001(2018).
[23] D. B. Straus, S. Hurtado Parra, N. Iotov, J. Gebhardt, A. M. Rappe, J. E. Subotnik, J. M. Kikkawa, C. R. Kagan. Direct observation of electron-phonon coupling and slow vibrational relaxation in organic-inorganic hybrid perovskites. J. Am. Chem. Soc., 138, 13798(2016).
[24] Z. Dang, Y. Luo, X.-S. Wang, M. Imran, P. Gao. Electron-beam-induced degradation of halide-perovskite-related semiconductor nanomaterials. Chin. Opt. Lett., 19, 030002(2021).
[25] B. Dhanabalan, Y. C. Leng, G. Biffi, M. L. Lin, P. H. Tan, I. Infante, L. Manna, M. P. Arciniegas, R. Krahne. Directional anisotropy of the vibrational modes in 2D-layered perovskites. ACS Nano, 14, 4689(2020).
[26] B. Robert. Resonance Raman spectroscopy. Photosynth. Res., 101, 147(2009).
[27] H. Hashimoto, Y. Koyama. Raman spectra of all-trans-β-carotene in the S1 and T1 states produced by direct photoexcitation. Chem. Phys. Lett., 163, 251(1989).
[28] X. Shen. Semiconductor Spectrum and Optical Properties,, 553(2002).
[29] P. Verma, S. C. Abbi, K. P. Jain. Raman-scattering probe of anharmonic effects in GaAs. Phys. Rev. B, 51, 16660(1995).
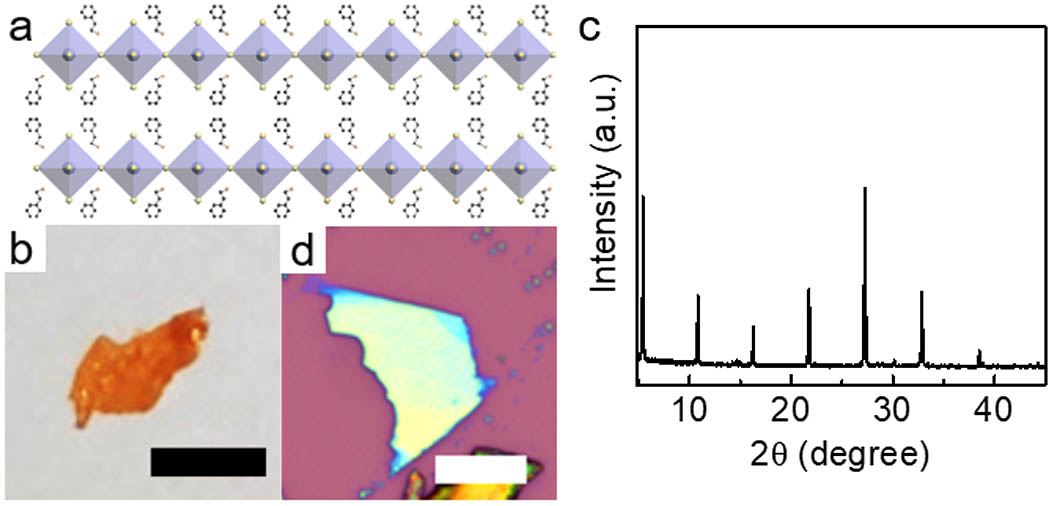
Set citation alerts for the article
Please enter your email address