Abstract
An all-optically reconfigurable generation of optical vortices would be highly beneficial to the implementation of next-generation optical communication and advanced information processing. The previously demonstrated approaches based on the parametric nonlinear optical processes, however, have exhibited limited conversion efficiency due to the group velocity mismatch and nonlinear phase shifts, and require the cumbersome preparation of either the optical element or initial seed beam having a non-zero topological charge. Here, we propose and analyze a novel scheme for highly efficient all-optical generation and control of optical vortices based on the dynamic acoustic vortex grating created by forward stimulated intermodal Brillouin scattering in a subwavelength-hole photonic waveguide. The dual-frequency pump beams in two different hybrid optical modes drive an acoustic vortex mode, which transforms a signal in the fundamental optical mode into an optical vortex mode. This scheme not only eliminates the need for the initial preparation of an angular-momentum-carrying medium or an optical vortex seed but also guarantees high modal purity and nearly 100% conversion efficiency assisted by the energy-momentum conservation. We also investigate the feasibility and practicability of the subwavelength-hole waveguides by examining the intermodal conversion efficiency and robustness of guidance of the optical vortices, taking into account the impact of the Kerr-type nonlinear effects on the intermodal Brillouin interactions based on our rigorous full-vectorial analytical theory.1. INTRODUCTION
Spatially structured light—an optical wave with a customized transverse profile of intensity, polarization, and phase—has been in the limelight as a versatile tool for exploring fundamental science and applications such as micro/nano-fabrication [1], topological statistics [2], astronomy [3], imaging [4], optical tweezers [5], quantum optics [6], and optical communications [7]. In particular, optical vortex beams (OVBs) possessing phase singularity at the beam center and a donut-shaped intensity pattern exhibit extraordinary properties that lead to new types of light–matter interactions, which in some cases enhance the performance of photonic devices and make a technological breakthrough. A notable example is a radical improvement in super-resolution fluorescence microscopy [4], where the conventional resolution limit can be circumvented by adopting the donut-shaped laser beam to cause the fluorescent molecules to be depleted only around the dark hollow beam center and leave a tiny beam spot from the fluorophore. Furthermore, helically phased light beams carry orbital angular momentum (OAM), which has been utilized mainly for exerting a torque precisely on the micro/nano-particles trapped in optical tweezers [5]. The optical OAM also provides intrinsically infinite dimensionality, bringing ongoing attention to high-dimensional quantum cryptography [6]. Mode-division multiplexing based on the OAM modes has also emerged as a scintillating solution to the exponentially growing demand for data capacity in optical communications [7].
Until very recently, OAM-carrying OVBs have been generated in most cases using specially designed optical media having topological charges, e.g., spiral phase plates [8], liquid crystal -plates [9], fiber gratings [10], and helically twisted fibers [11]. In addition, the development of holographic techniques using digital spatial light modulators (SLMs) has allowed for OVB generation based on real-time computer-controlled wavefront shaping [12]. On the other hand, parametric nonlinear optical processes have also attracted ongoing attention as an alternative means to generate and amplify the OVBs, as they enable dynamic all-optical manipulation and offer high damage threshold compared to the SLMs. All-optical creation of the OAM states has been relentlessly investigated using second-harmonic generation [13], intermodal four-wave mixing (FWM) [14], and parametric down-conversion [15], which would be beneficial especially to next-generation ultrahigh-capacity optical communications. However, these schemes have suffered from several disadvantages that hinder efficient OVB generation. The group velocity mismatch between the pump and signal gives rise to their temporal walk-off, which reduces the interaction length. In addition, the undesired nonlinear phase shift induced by the self-phase modulation (SPM) and cross-phase modulation (XPM) also limits the overall efficiency of the OVB generation. A multi-level atomic ensemble with a photonic bandgap structure has also been studied for transferring and controlling the OVBs via FWM and six-wave mixing, which could be modulated by superimposing the nonlinear phase shift in the atomic ensemble [16–19]. In this case, the interactions between the atomic ensemble and the electromagnetic fields could enhance the nonlinear optical susceptibility through the induced atomic coherence and the decrease of optical group velocity. However, they are not all-optical in the true sense because an optical seed carrying a topological charge is still required for their operation and therefore should be initially prepared using a delicately structured medium.
Meanwhile, the interaction between light and acoustic phonons has offered fundamentally different concepts of all-optical signal processing, as it has no all-optical analog among the parametric nonlinear optical processes. For instance, new optical frequencies or spatial modes can be excited efficiently through strong photon–phonon interactions even in the absence of optical resonances, which has been demonstrated in various forms, e.g., cascaded stimulated Raman-like scattering [20], phonon-mediated optical signal transmission [21], and unidirectional interband modulation [22]. The amplification and generation of OAM-carrying light via stimulated Raman scattering and stimulated Brillouin scattering (SBS) has been recently studied in liquid [23], gas [24], and plasmas [25]. On the other hand, in solid-state photonic waveguides such as optical fibers, it has only been demonstrated to date that the angular momentum in the externally applied acoustic wave can be transferred to the guided optical mode via acousto-optic interactions [26]. If the acoustic phonons could be optically generated and controlled in photonic waveguides, they would furnish promising techniques in photonic integrated circuits for all-optical manipulation of OAM light.
Sign up for Photonics Research TOC. Get the latest issue of Photonics Research delivered right to you!Sign up now
In this paper, we show that nonlinear optoacoustic interactions via forward stimulated intermodal Brillouin scattering (FSIBS) in suitably designed photonic waveguides can be employed for all-optical generation and control of OAM-carrying optical vortex modes (OVMs), which excludes the need for the initial preparation of any vortex seed. As shown in the schematic diagram of Fig. 1(a), when dual-frequency optical pump waves are launched into the waveguide, one frequency () being in the circularly polarized fundamental mode (i.e., ) and the other () in the higher-order hybrid mode (e.g., ), their beat produces oscillating optical forces (i.e., electrostrictive force and radiation pressure), which in turn coherently drive two orthogonal acoustic resonance (AR) modes of the frequency . The phases of the two AR modes differ from each other by 90°, creating an acoustic vortex mode (AVM) [26]. The optical signal of the frequency in the circularly polarized fundamental mode is then converted into the frequency-downshifted () targeted OVM via interaction with the AVM. We note that the intermodal coupling through the optically generated acoustic vortex grating might be related to the conventional backward-SBS-based dynamic grating in polarization-maintaining fibers, where a traveling acoustic wave created by two counterpropagating optical pump waves of one polarization reflects the orthogonally polarized signal [27]. It should be emphasized that in sharp contrast to the previously studied SBS-based mode conversion [28], in our case an optical signal in the circularly polarized fundamental mode is scattered into the associated OVM rather than the higher-order hybrid mode, the same as the higher-order pump mode, due to the total energy-momentum conservation. Hence, our scheme in principle guarantees high-purity OVM generation and can be exploited to switch the OVM state simply by varying the polarization state of the pump wave.
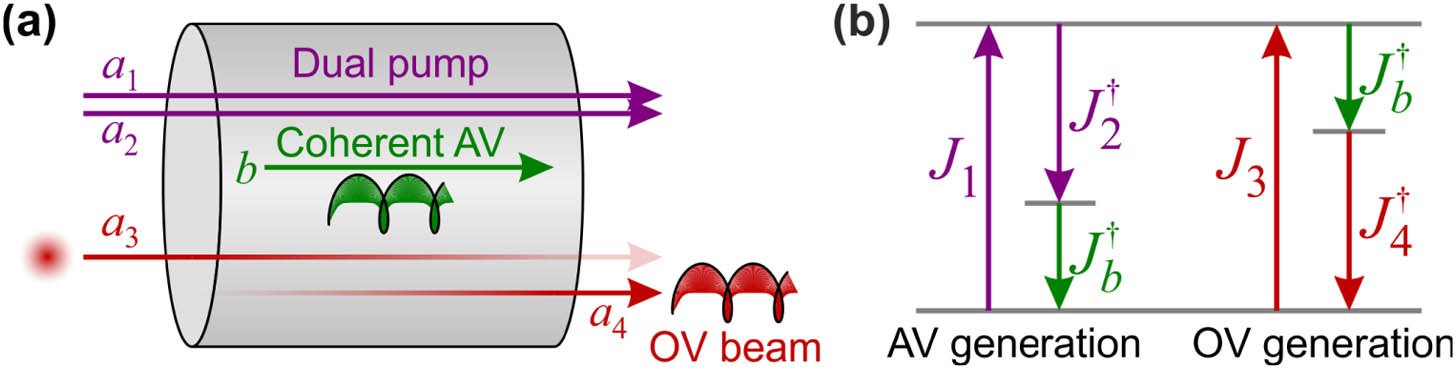
Figure 1.(a) Schematic diagram of optical vortex (OV) generation based on forward stimulated intermodal Brillouin scattering. A circularly polarized fundamental mode () and a higher-order hybrid mode () drive a coherent acoustic vortex (AV) (), which in turn transforms the incident signal () in the circularly polarized fundamental mode into the OV beam (). (b) Vector diagram describing the angular momentum conservation in this process.
While such a photon–phonon interaction is generally construed as a nonlinear wave-mixing process, it can also be described as an angular momentum transfer process between photons and phonons [Fig. 1(b)]. For paraxial beams propagating in free space or weakly guiding optical fibers, the spin angular momentum (SAM) and OAM are individually conserved in the photon–phonon interactions [23,25]. The AVM, having no SAM, can then convey to photons with its OAM only, their polarization state being unchanged. On the other hand, in strongly confining optical waveguides (e.g., microfibers, small-solid-core photonic crystal fibers, and on-chip integrated waveguides), the significant electric fields at the waveguide boundaries yield spin-orbit coupling [29]. In this case, the optical modes can no longer be treated as the eigenstates of either the SAM () or the OAM () operator, and the total angular momentum (AM) should be a conserved quantity. In sharp contrast to the OAM transfer processes in the paraxial regime [23,25], it turns out that both the SAM and OAM of the photons can be converted simultaneously in the FSIBS-based OVM generation process. This result would lead to a number of fundamentally intriguing and intricate phenomena in combination with the distinct features of optical AM, e.g., quantum spin Hall effect of light and optical spin-momentum locking [30].
2. PRINCIPLES
Here, we consider a silica-glass subwavelength-hole waveguide (SWHW) suspended in the air, as shown in Fig. 2(a), which has been proposed for robust OVM guidance against perturbations (e.g., bends and twists) [31] and can be implemented in the form of photonic crystal fiber having a circular air hole at the core center [32]. We numerically solve the eigenequations for both the optical and acoustic modes of the three-layered circularly symmetric SWHW [33,34]. We obtain the optical intensity profiles and phase distributions for the three lowest-order OVMs and express each of them in terms of the conventional hybrid modes, as summarized in Fig. 2(b). In general, the OVM, , can be represented as a linear combination of the corresponding even and odd hybrid modes with the phase difference of 90°, i.e., where (=+, −) and () denote the SAM and OAM quantum numbers, respectively, in the paraxial approximation [26], and is the radial mode number. For consistency, we designate the circularly polarized fundamental mode as the mode, although it is not an OVM but carries only an SAM.
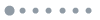
Figure 2.(a) Silica-glass subwavelength-hole waveguide (SWHW) suspended in the air, together with the Cartesian coordinates. (b) Intensity profiles of the circularly polarized fundamental mode and the three lowest-order optical vortex modes (OVMs), together with the corresponding compositions of the conventional even/odd hybrid modes for , , and . The light blue arrows represent the electric field distributions for the hybrid modes and the polarization states of the transverse electric fields for the OVMs. The rightmost column corresponds to the phase pattern of the component of the electric field, .
We determine the AVMs that are phase-matched with the optical modes by taking into account the dispersion of several acoustic modes [Fig. 3(a)]. For a given pair of the core radius and hole radius , we find an AVM satisfying the phase-matching condition for the two pump beams, where and are the axial wavevectors of the AVM and the two pump optical modes, respectively. The AVM, , carrying a topological charge of , can be expressed as a linear combination of the two degenerate conventional acoustic modes having an azimuthal mode number of with a 90° phase difference [26]. For example, the mode consists of the two orthogonal flexural modes and (“C” in Fig. 3) as , while the mode is comprised of the two axially anti-symmetric torsional-radial modes as (“D” in Fig. 3). (See Visualization 1 and Visualization 2 that display the vibrational motions of the and the modes, respectively.) We also consider the axially symmetric radial mode with no azimuthal dependence (), which we designate simply as the mode (“B” in Fig. 3). We note that we exclude the longitudinal mode (“A” in Fig. 3) in our analysis, as it is not efficiently driven by quasi-transverse optical forces that can yield the forward intermodal coupling.
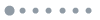
Figure 3.(a) Dispersion curves for the acoustic modes with the lowest radial mode number () in silica-glass SWHWs. The red, purple, and blue curves represent the acoustic modes with the three lowest azimuthal mode numbers, , 1, and 2, respectively. The frequencies and wavevectors of the acoustic modes are in units of and , respectively, where is the longitudinal sound velocity in fused silica. Some examples of the phase-matched acoustic modes are marked with green filled circles. (b) Displacement distributions of the longitudinal (A), radial (B), flexural (C), and torsional-radial (D) acoustic modes, together with the resulting acoustic vortex modes (AVMs). The solid and dashed curves represent the deformed and undeformed boundaries, respectively, and the color map is used to describe the profile of the magnitude of total displacement. All the calculations in (a) and (b) are carried out at the wavelength of and for the SWHW parameters of and . See Visualization 1 and Visualization 2, which display the vibrational motions of the and modes, respectively.
We model the propagation of the optical and acoustic modes as well as their interactions using the coupled-mode theory, under the viewpoint that each of the two pairs of optical modes—the two pump waves ( and ) and the incident/coupled signals ( and ) is coupled via the FSIBS-induced acoustic vortex grating () (Fig. 1). We also take into account the possibility that these interactions can be significantly influenced by the optical Kerr effect, as in the previously reported phase-locked Brillouin frequency comb generation in a nonlinear waveguide cavity that was affected by the Kerr-induced FWM [35]. Even in the absence of an optical cavity, the SPM and XPM can also make a discernible impact on the dynamics of the SBS-based wave-mixing process [36,37]. Furthermore, the nondegenerate intermodal FWM of the two pump waves and the incident signal may not only affect the targeted OVM signal () but also create an unwanted idler wave () in another OVM that is degenerate with the converted signal () but has the opposite total AM quantum number, . In our case, this idler wave gives rise to the detriment of the modal purity of the OVM signal, and therefore it is necessary to examine how significantly the idler wave affects the entire process. Taking all these into consideration, we derive the following steady-state () coupled-mode equations for the slowly varying amplitudes of the -th (, 2, 3, 4, 5) optical mode, , and the AVM, : where and represent the frequencies of the -th optical mode and the AVM, respectively, is the acoustic decay rate, and are the optoacoustic overlap integrals [38,39], and , , and are the nonlinear coefficients associated with the SPM, XPM, and Kerr-induced FWM, respectively [40]. We also introduce the wavevector mismatch , where are the axial wavevectors of the incident and coupled signal modes, while keeping the frequency condition .
3. RESULTS AND DISCUSSION
In our scheme, while the pump wave is kept in the () mode, the other can be in any higher-order hybrid mode, which determines the generated OVM according to the total AM conservation. For example, the FSIBS process with the and the modes creates the AVM having the total AM , i.e., the mode, where and are the total AM of the pump beam and one of the two constituent OVMs of the pump beam , the mode, respectively. The other constituent OVM of , the mode, does not participate in the optoacoustic interaction due to the energy-momentum conservation. The mode converts the signal beam in the mode with into the mode with .
Here, we focus on the AM transfer processes for the three lowest higher-order hybrid pump modes, the , , and modes, which we denote as PROC1, PROC2, and PROC3, respectively. To figure out which type of AVM can mediate each AM transfer process efficiently, we obtain and analyze the optical force distribution generated by the two pump beams, as illustrated in Fig. 4. In all the three cases, the total optical force is a linear combination of the two orthogonal force components with the phase difference of 90° and thus can drive the two orthogonal acoustic modes, which composes an AVM. In PROC1, since the and modes have the same (opposite) symmetry with respect to the () plane, the resulting optical force is symmetric (anti-symmetric) with respect to the () plane [Figs. 4(a) and 4(b)]. Then, the mode having the same symmetry with the azimuthal mode number can be excited. Similarly, the optical force induced by the and modes can drive the orthogonal mode, where the minus sign indicates that the optical force is anti-aligned with the displacement of the mode [Figs. 4(c) and 4(d)]. This reversal of the optical force direction can be seen from the fact that by rotating the x–y coordinates by 90°, one can find the phase difference of 180° between the optical force created by the and modes and that by the and modes. Since the two orthogonal flexural modes have a phase difference of 90°, the mode can be excited. A similar explanation holds for PROC3. In PROC2, on the other hand, the () and modes have the same (opposite) symmetries with respect to both the and planes. We note that in Fig. 4, we choose a particular global phase in such a way that the longitudinal component of the total electric field in the waveguide is purely real. Then, only the real part of the optical force spatially matches the profile of the mode, whereas the imaginary part that is anti-symmetric with respect to both planes does not have any effect on the generation of the mode.
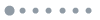
Figure 4.Real and imaginary parts of the typical optical force distributions for the three types of angular momentum (AM) transfer processes, PROC1, PROC2, and PROC3, which involve different higher-order hybrid optical modes: , , and modes, respectively. All the calculations are performed at the wavelength of and for the SWHW parameters of and . In addition, the photoelastic coefficients are set as and . For the electrostriction body force, the blue color intensities and black arrows depict the magnitude and direction, respectively, of the optical force. The optical boundary force is composed of the electrostriction force (blue) and radiation pressure (red), where their magnitude and direction are plotted by the arrows in the same scale for all the three processes for comparison. We choose a particular global phase in such a way that the longitudinal component of the total electric field in the waveguide is purely real.
We investigate the feasibility of our scheme of the optoacoustic OVM generation at the telecom wavelength () and assess the stability of guidance of the OVMs in silica-glass SWHWs. For typical mode conversion via FSIBS, in Eq. (2), because the two pairs of optical modes are set equal to each other ( and ) [41]. In this case, the input powers of the two pump beams should be strongly unbalanced to obtain the 100% mode conversion from to . However, this requires a very long waveguide for building up the acoustic amplitude significantly. In strong contrast, in our case of the OVM generation, the optoacoustic overlap integral between the two pump beams is exactly half of that involving the OVM because the OVM, , is a linear combination of the two hybrid optical modes as Eq. (1). As a result, 100% conversion from to can be achieved without the need for the unbalanced pump powers and impractically long interaction lengths. To quantitatively evaluate the feasibility, we define 3 dB coupling length, , as the propagation length over which half the input signal power is converted into the targeted OVM or the idler wave (i.e., the other constituent OVM) at balanced input powers of the two pump beams, i.e., , where is the input power of the -th optical wave. To examine the robustness of the OVM transmission in the waveguide, we check the two following requirements. First, it is essential to have a sufficiently large effective index separation between the adjacent optical modes of the same OAM order. In our case, this requirement is ensured by the large index step between the silica ring and air [42]. It is widely accepted that the effective index separation of is enough for stable OVM propagation, where is defined as the effective index difference between the adjacent vector modes, e.g., among the , , and modes for PROC1, and between the and modes for PROC2 and PROC3. In addition, the generated OVMs should possess high modal purity and low propagation loss simultaneously. Here, we define the modal purity as the power portion of the desired OVM in the converted signal wave, i.e., . We estimate the modal power fraction confined in the core (), which is in practice related to the energy leakage into the cladding of the photonic crystal fibers [43]. The larger modal power fraction leads to a lower propagation loss.
We calculate , , and for the three AM transfer processes over a range of the core radius and the radius ratio . In all the following analyses, for simplicity, we fix the acoustic quality factor as 1000 for fused silica glass [44] and the total pump power as . The larger and smaller yield a larger power fraction in the core. It turns out that in the silica-glass SWHWs with , the Kerr nonlinear coefficients, , , and , are estimated below , whereas the FSIBS peak gain coefficient is much larger than them, e.g., for PROC1 and for PROC2 and PROC3, so the optical Kerr effect is negligible in Eq. (2). The modal purity of the generated OVM is then almost 100%, as the FWM-induced generation of the idler wave is suppressed. On the other hand, in the SWHWs made of a higher-index material like silicon, the Kerr nonlinear coefficients become comparable to the FSIBS peak gain coefficient, and thus the optical Kerr effect cannot be ignored, which we will not discuss here in more detail. In PROC1 [Fig. 5(a)], a large effective index separation can be achieved over a wide range of (). As the radius ratio rises, the radiation pressure on the silica–air boundaries gets enhanced and more dominant in the FSIBS process, and thus gets shorter at the expense of the transmission loss. The 3 dB mode conversion occurs typically at propagation over a broad range of (), which is short enough to implement with the current fabrication technologies. In PROC2 and PROC3, we can also find the range of () suitable for our scheme. However, the range of useful waveguide parameters is not so wide compared to the PROC1 case, because the typical value of is on the order of hundreds of meters. decreases as falls, and should be small enough () in order to obtain sufficiently small values of . Moreover, between the and the modes is relatively small and can be even zero. We note that at certain waveguide parameters, the FSIBS can be completely suppressed due to the counterbalance between the electrostriction and radiation pressure [38,39], as displayed by red striped regions, i.e., and for PROC2 in Fig. 5(b) and and for PROC3 in Fig. 5(c). We highlight that we here report for the first time, to our knowledge, the case of FSIBS elimination, while the cancellation of the conventional backward surface acoustic wave Brillouin scattering and forward intramodal (Raman-like) Brillouin scattering has been recently reported in Refs. [45,46] and Ref. [47], respectively.
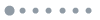
Figure 5.Color maps displaying the 3 dB coupling length in silica-glass SWHWs over a range of and for (a) PROC1, (b) PROC2, and (c) PROC3, where the pink curves indicate the cutoff of the , , and mode, respectively. The contour plots on top of the color maps show the minimum between the adjacent vector modes, i.e., among the , , and modes for (a), and between the and modes for (b) and (c). The black curves correspond to the power fraction in the waveguide core. The OVMs have lower confinement losses over the regions below the black curves.
We then investigate the spatial evolution of each optical wave. For simplicity, we set the waveguide parameters as , at which for PROC1 the effective index separation, power fraction in the core, FSIBS peak gain coefficient, and 3 dB coupling length are calculated as , , , and , respectively, and the Kerr nonlinear coefficients (, , and ) are in the range of . Here, we use the long-waveguide approximation, assuming that the acoustic modes are damped more rapidly than the optical pump power changes [38]. This assumption is valid because the acoustic decay length () is smaller than . We calculate the spectral dependence of the mode conversion efficiency, , over the entire range of the input pump power , while fixing the total pump power at and the propagation length at 3 m. Here, we set the frequency difference between the two pump beams ( and ) as well as between the incident and coupled signals ( and ) to exactly match the acoustic frequency . We determine the wavevector mismatch considering the optical dispersion of the silica-glass SWHWs. As previously mentioned, unity conversion efficiency can be obtained at [Fig. 6(a)]. At this balanced pump-power condition, the full width at half maximum (FWHM) operating bandwidth for the signal is determined as 26 GHz (0.21 nm) from Fig. 6(b). We can further optimize the waveguide length by setting the two input pump powers unbalanced (). We can in general think of two regimes of the input pump power ratio , as shown in Fig. 6(c). When , the acoustic power is concentrated at the beginning of the waveguide section, which leads to insufficient coupling strength for full mode conversion. On the other hand, when , sufficient acoustic power can be sustained over a longer section of the waveguide to even over-couple the signal mode. In this case, it is possible to control the waveguide length to achieve unity conversion. We optimize the waveguide length and recalculate in Fig. 6(d), where we find the maximum FWHM operating bandwidth of 50 GHz (0.40 nm) at .
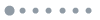
Figure 6.Spatial evolution of the optical and acoustic powers in PROC1 in silica-glass SWHW with . (a) Optical and acoustic powers as functions of the propagation distance, , at zero signal frequency detuning . and are normalized to , and to , and to . (b) Mode conversion efficiency after propagation along , where the optical dispersions of the silica-glass SWHWs are taken into account. (c) Same plots as (a) but at the different signal frequency detuning with different input pump power ratios of (dashed curves) and (solid curves). (d) Mode conversion efficiency at the optimum waveguide length , where is the propagation length at which is maximized.
The theory we have developed so far can be applied to any types of nonlinear intermodal optoacoustic coupling and arbitrary waveguide cross-sectional geometries. In particular, recent studies suggest that such nonlinear intermodal coupling could also be implemented in on-chip integrated waveguides, which can be fabricated using the standard CMOS-compatible technology [22,28]. While our approach of OVM generation has a unique advantage over other kinds of nonlinear optical methods, i.e., no initial OVM seed is required, there are still some important aspects that should be further addressed to put our scheme into practical use. First, it is essential to ensure that the hybrid modes are well controlled and generated. Mode converters would be needed at the input of the waveguide to couple light into the higher-order hybrid modes. Also, the mode filtering is required to separate the generated OVM () from the residual fundamental mode (). We note that various types of multimode waveguide-based photonic devices [48,49] would provide possible solutions for this purpose, which have been experimentally demonstrated in the fiber [50] and integrated waveguide platform [51]. For example, the multimode Y-junctions based on the adiabatic evolution of effective modal indices can be used as mode combiners/splitters and mode converters [49]. Another issue is the narrow operating spectral bandwidth, as shown in Fig. 6. The limited bandwidth is due to the group velocity difference between the two hybrid modes, e.g., the and the modes, which increases the wavevector mismatch with the detuning of signal wavelength from the pump wavelength. While it is not straightforward to match the dispersions of the two optical modes in simple step-index SWHWs, in photonic crystal fibers, the group velocities of the two different optical modes can be made equal to each other by adequately adjusting the pitch and hole size in the cladding [52]. The operating spectral bandwidth would be then significantly improved, potentially beyond [52]. One might think that the strong pump beams may give rise to the temperature increase of the waveguide, which alters the effective indices of the optical modes. However, we determine from the heat diffusion analysis [53] that the influence of such a thermo-optic effect is not significant in practical waveguides such as silica-glass photonic crystal fibers [32], as the temperature increase is far below 1°C at the 1 W pump power. Finally, the robust guidance of the hybrid modes in optical waveguides is impeded by the random linear mode coupling due to the residual defects and random birefringence fluctuations.
4. CONCLUSION
In conclusion, nonlinear optoacoustic interactions via FSIBS can be employed for the generation of OAM-carrying optical vortices, which has a unique advantage of excluding the need for any initial optical vortex seed. We have presented numerical results of the intermodal coupling involving the OVM and the AVM, and it turns out that a waveguide with a subwavelength-hole of proper diameter at the core center is suitable for not only the robust guidance but also the efficient optoacoustic generation of the OVMs. We point out that the FSIBS process is the right nonlinear effect for all-optical OVM generation, where nearly unity conversion efficiency and high modal purity are guaranteed with the aid of the energy-momentum conservation. Our scheme can be realized in various optical systems, such as microstructured fibers, microcavities, and integrated optical circuits.
Our findings open an avenue for providing a key technology for next-generation ultrahigh-bandwidth optical communication systems. For instance, OAM-selective photon–phonon interactions could be employed to fabricate the spatial mode multiplexers [7]. We have shown that coupling with the optoacoustically generated AVM enables the selective excitation of one of the constituent OVMs of the pump beams. Such all-optical control of the AVMs in the waveguides might also be exploited for the application of axial torque to axisymmetric objects and the transport of modulated helicity [54]. Furthermore, introducing AM transfer to the intermodal coupling allows us to generate and manipulate non-classical correlations between OAM-carrying photons and phonons, and to explore new types of quantum phenomena [55].
References
[1] K. Toyoda, K. Miyamoto, N. Aoki, R. Morita, T. Omatsu. Using optical vortex to control the chirality of twisted metal nanostructures. Nano Lett., 12, 3645-3649(2012).
[2] M. R. Dennis, R. P. King, B. Jack, K. O’Holleran, M. J. Padgett. Isolated optical vortex knots. Nat. Phys., 6, 118-121(2010).
[3] G. Foo, D. M. Palacios, G. A. Swartzlander. Optical vortex coronagraph. Opt. Lett., 30, 3308-3310(2005).
[4] S. W. Hell, J. Wichmann. Breaking the diffraction resolution limit by stimulated emission: stimulated-emission-depletion fluorescence microscopy. Opt. Lett., 19, 780-782(1994).
[5] H. He, M. E. J. Friese, N. R. Heckenberg, H. Rubinsztein-Dunlop. Direct observation of transfer of angular momentum to absorptive particles from a laser beam with a phase singularity. Phys. Rev. Lett., 75, 826-829(1995).
[6] M. Mirhosseini, O. S. Magaña-Loaiza, M. N. O’Sullivan, B. Rodenburg, M. Malik, M. P. J. Lavery, M. J. Padgett, D. J. Gauthier, R. W. Boyd. High-dimensional quantum cryptography with twisted light. New J. Phys., 17, 033033(2015).
[7] N. Bozinovic, Y. Yue, Y. Ren, M. Tur, P. Kristensen, H. Huang, A. E. Willner, S. Ramachandran. Terabit-scale orbital angular momentum mode division multiplexing in fibers. Science, 340, 1545-1548(2013).
[8] M. W. Beijersbergen, R. P. C. Coerwinkel, M. Kristensen, J. P. Woerdman. Helical-wavefront laser beams produced with a spiral phaseplate. Opt. Commun., 112, 321-327(1994).
[9] L. Marrucci, C. Manzo, D. Paparo. Optical spin-to-orbital angular momentum conversion in inhomogeneous anisotropic media. Phys. Rev. Lett., 96, 163905(2006).
[10] H. Xu, L. Yang. Conversion of orbital angular momentum of light in chiral fiber gratings. Opt. Lett., 38, 1978-1980(2013).
[11] C. N. Alexeyev, M. A. Yavorsky. Generation and conversion of optical vortices in long-period helical core optical fibers. Phys. Rev. A, 78, 043828(2008).
[12] A. W. Lohmann, D. P. Paris. Binary Fraunhofer holograms, generated by computer. Appl. Opt., 6, 1739-1748(1967).
[13] K. Dholakia, N. B. Simpson, M. J. Padgett, L. Allen. Second-harmonic generation and the orbital angular momentum of light. Phys. Rev. A, 54, R3742-R3745(1996).
[14] W. Jiang, Q. F. Chen, Y. S. Zhang, G. C. Guo. Computation of topological charges of optical vortices via nondegenerate four-wave mixing. Phys. Rev. A, 74, 043811(2006).
[15] J. Arlt, K. Dholakia, L. Allen, M. J. Padgett. Parametric down-conversion for light beams possessing orbital angular momentum. Phys. Rev. A, 59, 3950-3952(1999).
[16] Y. Zhang, Z. Nie, Y. Zhao, C. Li, R. Wang, J. Si, M. Xiao. Modulated vortex solitons of four-wave mixing. Opt. Express, 18, 10963-10972(2010).
[17] Z. Zhang, D. Ma, Y. Zhang, M. Cao, Z. Xu, Y. Zhang. Propagation of optical vortices in a nonlinear atomic medium with a photonic band gap. Opt. Lett., 42, 1059-1062(2017).
[18] D. Zhang, X. Liu, L. Yang, X. Li, Z. Zhang, Y. Zhang. Modulated vortex six-wave mixing. Opt. Lett., 42, 3097-3100(2017).
[19] Z. Wang, J. Yang, Y. Sun, Y. Zhang. Interference patterns of vortex beams based on photonic band gap structure. Opt. Lett., 43, 4354-4357(2018).
[20] M. S. Kang, A. Nazarkin, A. Brenn, P. St.J. Russell. Tightly trapped acoustic phonons in photonic crystal fibres as highly nonlinear artificial Raman oscillators. Nat. Phys., 5, 276-280(2009).
[21] H. Shin, J. A. Cox, R. Jarecki, A. Starbuck, Z. Wang, P. T. Rakich. Control of coherent information via on-chip photonic-phononic emitter-receivers. Nat. Commun., 6, 6427(2015).
[22] E. A. Kittlaus, N. T. Otterstrom, P. Kharel, S. Gertler, P. T. Rakich. Non-reciprocal interband Brillouin modulation. Nat. Photonics, 12, 613-619(2018).
[23] W. Gao, C. Mu, H. Li, Y. Yang, Z. Zhu. Parametric amplification of orbital angular momentum beams based on light-acoustic interaction. Appl. Phys. Lett., 107, 041119(2015).
[24] M. F. Andersen, C. Ryu, P. Cladé, V. Natarajan, A. Vaziri, K. Helmerson, W. D. Phillips. Quantized rotation of atoms from photons with orbital angular momentum. Phys. Rev. Lett., 97, 170406(2006).
[25] J. T. Mendonça, B. Thidé, H. Then. Stimulated Raman and Brillouin backscattering of collimated beams carrying orbital angular momentum. Phys. Rev. Lett., 102, 185005(2009).
[26] P. Z. Dashti, F. Alhassen, H. P. Lee. Observation of orbital angular momentum transfer between acoustic and optical vortices in optical fiber. Phys. Rev. Lett., 96, 043604(2006).
[27] K. Y. Song, W. Zou, Z. He, K. Hotate. All-optical dynamic grating generation based on Brillouin scattering in polarization-maintaining fiber. Opt. Lett., 33, 926-929(2008).
[28] E. A. Kittlaus, N. T. Otterstrom, P. T. Rakich. On-chip inter-modal Brillouin scattering. Nat. Commun., 8, 15819(2017).
[29] J. Petersen, J. Volz, A. Rauschenbeutel. Chiral nanophotonic waveguide interface based on spin-orbit interaction of light. Science, 346, 67-71(2014).
[30] K. Y. Bliokh, D. Smirnova, F. Nori. Quantum spin Hall effect of light. Science, 348, 1448-1451(2015).
[31] Y. Yue, L. Zhang, Y. Yan, N. Ahmed, J.-Y. Yang, H. Huang, Y. Ren, S. Dolinar, M. Tur, A. E. Willner. Octave-spanning supercontinuum generation of vortices in an As2S3 ring photonic crystal fiber. Opt. Lett., 37, 1889-1891(2012).
[32] G. S. Wiederhecker, C. M. B. Cordeiro, F. Couny, F. Benabid, S. A. Maier, J. C. Knight, C. H. B. Cruz, H. L. Fragnito. Field enhancement within an optical fibre with a subwavelength air core. Nat. Photonics, 1, 115-118(2007).
[33] K. Okamoto. Fundamentals of Optical Waveguides(2006).
[34] R. A. Waldron. Some problems in the theory of guided microsonic waves. IEEE Trans. Microwave Theory Tech., 17, 893-904(1969).
[35] T. F. S. Büttner, I. V. Kabakova, D. D. Hudson, R. Pant, C. G. Poulton, A. C. Judge, B. J. Eggleton. Phase-locking and pulse generation in multi-frequency Brillouin oscillator via four wave mixing. Sci. Rep., 4, 5032(2014).
[36] H. Shin, W. Qiu, R. Jarecki, J. A. Cox, R. H. Olsson, A. Starbuck, Z. Wang, P. T. Rakich. Tailorable stimulated Brillouin scattering in nanoscale silicon waveguides. Nat. Commun., 4, 1944(2013).
[37] R. Van Laer, B. Kuyken, D. Van Thourhout, R. Baets. Interaction between light and highly confined hypersound in a silicon photonic nanowire. Nat. Photonics, 9, 199-203(2015).
[38] C. Wolff, M. J. Steel, B. J. Eggleton, C. G. Poulton. Stimulated Brillouin scattering in integrated photonic waveguides: forces, scattering mechanisms, and coupled-mode analysis. Phys. Rev. A, 92, 013836(2015).
[39] P. T. Rakich, C. Reinke, R. Camacho, P. Davids, Z. Wang. Giant enhancement of stimulated Brillouin scattering in the subwavelength limit. Phys. Rev. X, 2, 011008(2012).
[40] S. Afshar, T. M. Monro. A full vectorial model for pulse propagation in emerging waveguides with subwavelength structures part I: Kerr nonlinearity. Opt. Express, 17, 2298-2318(2009).
[41] X. Huang, S. Fan. Complete all-optical silica fiber isolator via stimulated Brillouin scattering. J. Lightwave Technol., 29, 2267-2275(2011).
[42] S. Ramachandran, P. Kristensen, M. F. Yan. Generation and propagation of radially polarized beams in optical fibers. Opt. Lett., 34, 2525-2527(2009).
[43] H. Zhang, X. Zhang, H. Li, Y. Deng, X. Zhang, L. Xi, X. Tang, W. Zhang. A design strategy of the circular photonic crystal fiber supporting good quality orbital angular momentum mode transmission. Opt. Commun., 397, 59-66(2017).
[44] R. M. Shelby, M. D. Levenson, P. W. Bayer. Guided acoustic-wave Brillouin scattering. Phys. Rev. B, 31, 5244-5252(1985).
[45] O. Florez, P. F. Jarschel, Y. A. V. Espinel, C. M. B. Cordeiro, T. P. Mayer Alegre, G. S. Wiederhecker, P. Dainese. Brillouin scattering self-cancellation. Nat. Commun., 7, 11759(2016).
[46] D. S. Han, I.-M. Lee, K. H. Park, M. S. Kang. Extremely polarization-sensitive surface acoustic wave Brillouin scattering in subwavelength waveguides. Appl. Phys. Lett., 113, 121108(2018).
[47] D. S. Han, I.-M. Lee, K. H. Park, M. S. Kang. Polarization-selective control of nonlinear optomechanical interactions in subwavelength elliptical waveguides. Opt. Express, 27, 1718-1726(2019).
[48] L. B. Soldano, E. C. M. Pennings. Optical multi-mode interference devices based on self-imaging: principles and applications. J. Lightwave Technol., 13, 615-627(1995).
[49] J. D. Love, N. Riesen. Single-, few-, and multimode Y-junctions. J. Lightwave Technol., 30, 304-309(2012).
[50] R. Ismaeel, T. Lee, B. Oduro, Y. Jung, G. Brambilla. All-fiber fused directional coupler for highly efficient spatial mode conversion. Opt. Express, 22, 11610-11619(2014).
[51] L.-W. Luo, N. Ophir, C. P. Chen, L. H. Gabrielli, C. B. Poitras, K. Bergmen, M. Lipson. WDM-compatible mode-division multiplexing on a silicon chip. Nat. Commun., 5, 3069(2014).
[52] M. Bache, H. Nielsen, J. Lægsgaard, O. Bang. Tuning quadratic nonlinear photonic crystal fibers for zero group-velocity mismatch. Opt. Lett., 31, 1612-1614(2006).
[53] D. C. Brown, H. J. Hoffman. Thermal, stress, and thermo-optic effects in high average power double-clad silica fiber lasers. IEEE J. Sel. Top. Quantum Electron., 37, 207-217(2001).
[54] L. Zhang, P. L. Marston. Angular momentum flux of nonparaxial acoustic vortex beams and torques on axisymmetric objects. Phys. Rev. E, 84, 065601(2011).
[55] R. Riedinger, S. Hong, R. A. Norte, J. A. Slater, J. Shang, A. G. Krause, V. Anant, M. Aspelmeyer, S. Gröblacher. Non-classical correlations between single photons and phonons from a mechanical oscillator. Nature, 530, 313-316(2016).