Abstract
We systematically investigate the influences of the input infrared spectrum, chirp, and polarization on the emitted intense terahertz spectrum and spatial dispersion in lithium niobate via optical rectification. The terahertz yield and emission spectrum depend on both the chirp and spectrum of the input pump laser pulses. We also observe slight non-uniform spatial dispersion using a knife-edge measurement, which agrees well with the original predictions. The possible mechanism is the nonlinear distortion effect caused by high-energy laser pumping. Our study is very important and useful for developing intense terahertz systems with applications in extreme terahertz sciences and nonlinear phenomena.1. INTRODUCTION
Extreme terahertz science has become the second research hotspot in terahertz science and technology [1]. Along with the rapid development of strong-field terahertz sources [2,3], terahertz research has moved from the linear stage to the nonlinear stage with a variety of exciting applications, such as strong-field-induced phase transitions and new phenomena and physics in condensed matter, ultrafast terahertz spintronics, terahertz nonlinear optics, ultrafast thermochemical reaction kinetics, and terahertz biological effects [4–9]. Therefore, high-energy strong-field terahertz sources are in high demand. Among recently developed intense terahertz sources [10], ultrafast-femtosecond-laser-based intense terahertz sources are the most promising. Laser plasma-based terahertz sources are very promising because there is no damage threshold requirement [2]. However, solid-state intense terahertz sources, to some extent, have their unique merits, especially for real applications [6–9]. Among them, organic-crystal-based and lithium-niobate-crystal-based solid-state intense terahertz sources are the most outstanding. Recently, high-energy terahertz generation with 0.9 mJ pulse energy and 3% optical-to-terahertz energy conversion efficiency has been obtained in organic crystals via optical rectification [11]. However, scaling up this source to higher energy has met roadblocks, such as the low damage threshold of the organic crystal, its small size and high cost, and its specific wavelength pumping. These imperfections have hindered the development of organic-crystal-based intense terahertz sources. The other option for a solid-state intense terahertz source is a lithium-niobate-crystal-based source [12]. Thanks to the tilted pulse front technique, this kind of source can deliver intense terahertz pulses, which have been widely used in laboratories all over the world [13,14].
The tilted pulse front technique was first proposed and experimentally demonstrated in 2002 by Prof. Hebling [12]. During the intervening years, many world records, including best optical-to-terahertz energy conversion efficiency [15], highest output terahertz energy, and strongest terahertz fields, have been obtained [16–20]. People have systematically investigated the laser parameter influence on terahertz generation efficiency and have concluded that longer wavelengths as pumping pulses will have higher efficiency [21–26]. Longer pulse durations of 600 fs for a 1 μm laser wavelength are the most appropriate for terahertz generation, while 350 fs is best for 800 nm pumping [27–29]. In order to obtain a higher terahertz yield, for example, millijoule (mJ)-level terahertz generation requires sub-joule (J)/J-level pumping laser pulse energy. These kinds of laser systems are expensive and built for other applications with extremely short pulse durations of . Therefore, how to overcome the limitations of ultrashort pump laser pulses resulting in the decrease of the terahertz efficiency has become a key challenge [30]. One method is cutting the spectrum inside the laser compressor, resulting in longer pulse duration [31]. This method has the drawback of wasting a great deal of pump energy. The other approach is adding temporal chirp onto the pump laser pulses, resulting in lower pump peak intensity and longer effective interaction length [13]. However, when tuning the chirp of the pump laser pulses, the acousto-optic programmable dispersive filter (AOPDF, Dazzler, Fastlite) will also introduce the variation of the pump spectrum, which makes it difficult to distinguish the influence mechanism. Furthermore, when a high-energy pump laser is employed in the tilted pulse front technique, the nonlinear distortion effect has become obvious [22]. This means the generated terahertz beam position and spot size but also the output terahertz spectrum and its spatial frequency distribution are dependent on the pump energy, chirp, and other parameters. Due to the nonlinear geometrical optical setup, this phenomenon has become very important to understanding the generation theory. It should also be given attention when building intense terahertz application systems. However, these phenomena have not yet been systematically investigated both from the experimental and theoretical sides.
In this work, we experimentally study the impacts of the pump laser energy, polarization, and chirp output on the emitted terahertz spectrum and spatial frequency distribution. It includes the following parts: (i) the influence of the pump laser chirp and input spectrum on the terahertz efficiency and output spectrum; (ii) the influence of pump laser polarization on terahertz generation; (iii) the out-coupled terahertz spectrum as a function of the pump laser energy; (iv) the terahertz spatial chirp effect. The last paragraph is the conclusion and outlook.
Sign up for Photonics Research TOC. Get the latest issue of Photonics Research delivered right to you!Sign up now
2. EXPERIMENTAL SETUP
In our intense terahertz generation system, we employ a commercial Ti:sapphire laser amplifier system to pump the lithium niobate crystal. More details of the experimental setup can be found in Ref. [13]. The lithium niobate crystal used in our experiment is a -cut congruent crystal with the triangle prism shape. Its dimensions in the plane is , giving two 62° angles for terahertz generation. The height of the lithium niobate crystal in the axis is 40 mm, and the crystal is doped with 6.0 mol. % MgO in order to increase the damage threshold. It is anti-reflection (AR) coated in the 700–1060 nm wavelength range on two rectangular surfaces () to overcome the Fresnel losses. In order to study the influence of the laser chirp, energy, and polarization on the terahertz generation efficiency and spectrum, we introduce the second-order dispersion into the pump laser pulses. The transform-limited output pulse measured by frequency-resolved optical gating (FROG) is 30 fs. With the temporal chirp method, the pulse duration can be extended to several picoseconds with negative and positive chirp. With this method, we not only decrease the pump peak intensity, avoiding the crystal damage [21,31], but also increase the effective interaction length for longer phase matching, expecting higher terahertz generation efficiency. For the comparable study of the pump energy on the terahertz generation, we vary the pump laser energy before it enters the tilted pulse front setup, guaranteeing the polarization of the pump laser is kept constant, or rotate the half-wave plate between the grating and the crystal so that the pump energy is not varied and only the polarization is changed. We record the extracted terahertz energy with a pyroelectric detector and its corresponding spectrum via electro-optic sampling. For the measurement of terahertz spatial dispersion, we use a knife-edge measurement directly after the emission crystal, as shown in Fig. 1.
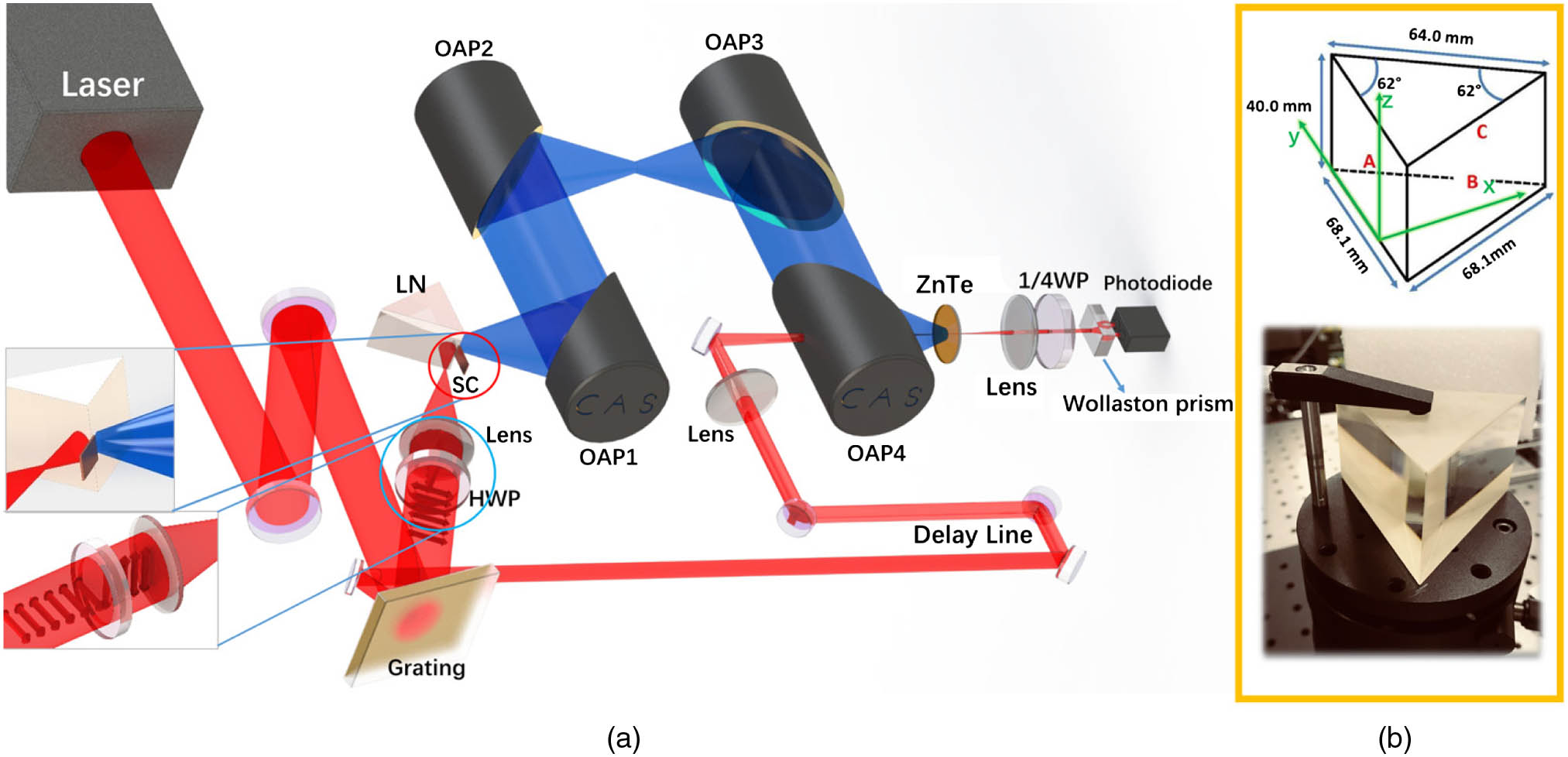
Figure 1.(a) Experimental setup for systematic characterization of the terahertz emission spectrum depends on the pump polarization, the emission position, as well as the input spectrum. The grating density is of 1500 lines/mm. The focal length of the imaging lens between the grating is 85 mm; HWP, half wave plate; SC, sheet copper; LN, lithium niobate crystal; OAP, 90° off-axis parabolic mirror; 1/4 WP, quarter-wave plate. (b) Photo of the lithium niobate crystal used in our experiment as well as its axis illustration.
3. RESULTS AND DISSCUSSION
In our previous work [13], we observed that the optical-to-terahertz energy conversion efficiency not only depends on the external applied group velocity dispersion (GVD) of the pump pulses but is also influenced by the infrared input spectrum. In this experiment, we further study the impact of the infrared pump spectrum on the emitted terahertz spectrum. Therefore, we record the infrared input spectrum of different chirped pulses with varied GVD shown in Fig. 2(a). It is obvious that the infrared input spectrum is strongly modulated by adding temporal chirp onto the pump pulses, resulting in the different terahertz yields shown in Fig. 2(c). The maximum terahertz signal of (160 μJ) is obtained when the GVD is (370 fs). Theoretical prediction has been demonstrated that the optimal pump pulse duration for 800 nm central wavelength pumping is [28]. For shorter pumping pulses, the effective interaction length is too short, and the pump peak intensity is too high. The combined effects are to reduce the effective interaction length and lead to multiphoton absorption for the pumping laser pulses. Both negative effects will decrease the optical-to-terahertz energy conversion efficiency. For the longer pump pulse duration, the peak intensity is low, which will also reduce the terahertz efficiency. In our case, since we are employing a high-energy Ti:sapphire laser system for the terahertz generation, the input pump pulse duration is , which is not only too short for effective interaction length, but also results in too-high pump intensity, leading to multiphoton absorption [21,31]. Therefore, extending the pump pulse duration by simply chirping it plays an important role in scaling up the terahertz yields [32–34]. For the GVD of , the input spectrum in Fig. 2(a) is relatively flat compared with others that have many peaks, especially for a GVD value larger than . For these chirped pulses with large GVD values, not only is the pump pulse duration too long, resulting in reduced pump intensity, but also the varied pump spectrum with different wavelength magnitude decreases the terahertz yields. Even the tilted pulse front setup is optimized for the best efficiency for different GVDs; the deposited angular dispersion is best optimized for the flat pump spectrum with its central wavelength of , which is designed for this wavelength. Figure 2(b) summarizes the measured terahertz emission spectra for different GVDs. Along with the increasing of the GVD value, the emission spectrum is broadened from 0.18 to 0.35 THz with a slight shift of the central frequency from to 0.31 THz. The variation of the output terahertz spectrum with the infrared input chirp and spectrum has not been systematically studied. For the generated terahertz spectrum width, due to the cascading process in the tilted pulse front technique, infrared photons will continuously downconvert to terahertz photons as long as the phase-matching condition is satisfied. Therefore, for transform-limited pulse pumping, a broader terahertz spectrum will be generated for shorter pulse duration of the pumping pulses. In our case, with different pump pulses with varied chirp and spectrum, the changed effective interaction length may introduce different propagation distances for the generated terahertz wave inside the lithium niobate crystal. The chirped pulses with larger GVDs correspond to longer pulse durations, which have lower pump intensity, resulting in lower generation efficiency. Thus, the infrared pump spectrum would not be strongly destroyed when it enters the crystal facet. When it propagates inside the crystal, it continues generating terahertz photons. Therefore, for the higher frequency component of the generated terahertz beam, it will propagate shorter distance and be absorbed less inside the crystal, resulting in a higher frequency out-coupled for larger GVD pump pulses. However, we only propose a qualitative explanation for the observed experimental phenomenon. Deep understanding of the physical process for the influence of the pump spectrum on the terahertz efficiency needs further theoretical investigation.
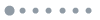
Figure 2.(a) Normalized infrared (IR) input spectra for different GVD, and (b) their corresponding output terahertz spectra. (c) The detected terahertz signal as a function of the different GVD values of the pump pulses; (d) terahertz bandwidth and terahertz central frequency as functions of GVD values of the pump pulses; (e) calculated chirped pulse duration as a function of the different GVD values of the pump pulses.
When high energy of pump pulses illuminates onto the lithium niobate crystal, the generated terahertz waves will be influenced by two effects. The first straightforward effect is optical heating, which increases the crystal temperature, resulting in the scaling up of the absorption for the generated terahertz waves. This is due to the linear absorption of crystal lattice motion, which absorbs more terahertz energy when the temperature increases. The other effect is nonlinear distortion due to the variation of the effective interaction length inside the crystals [22]. This effect will induce the change of the beam size, the relative emission position inside the crystal, and also its emission spectrum. Therefore, for real application systems in which the usable terahertz beam energy has to be varied, varying the pump energy is not a good solution to realize this goal. Both the optical heating and the nonlinear distortion effect are expected to happen when we change the pump energy. In order to overcome this problem, we here study the terahertz output energy as well as its spectrum as functions of the pump polarization. In our case, we keep the pump energy as constant of 86 mJ and vary its polarization. Since the optical axis of the lithium niobate crystal for highly efficient terahertz generation should be parallel to the pump polarization, there is a cosine relationship between terahertz output energy and the rotation angle of the infrared polarizer, which is consistent with the experimental result shown in Fig. 3(d). Surprisingly, it is observed that the detected terahertz signal is not symmetrically dependent on the polarization angle of the infrared pump pulses. From Fig. 3(d) we can see that the weakest terahertz signal appears at the rotation angle of . Facing the pump laser propagation direction, the detected terahertz signals for left-hand rotation are always larger than those obtained for right-hand rotation. This may be due to the fact that the magnitude of the second-order nonlinear coefficient in the two directions perpendicular to the optical axis is not the same, resulting in a weak component contribution of coherent generation coupled to the detected signal. Furthermore, we systematically characterize the output terahertz spectrum by measuring the electro-optic sampled temporal waveforms and then Fourier transform the signals from the time domain to the frequency domain. Figure 3(a) illustrates the recorded terahertz output spectra for different rotation angles of the infrared half-wave plate in the rotation angle ranges of 0°–80° and 100°–180°. In order to clarify the influence of the pump polarization on the terahertz spectrum, Fig. 3(b) shows the one-by-one comparison between several pairs of the corresponding angles. The slightly asymmetric influence between these polarization angles on the generated terahertz central frequency and spectral width is summarized in Fig. 3(c). As is shown in Fig. 3(c), there is no big impact of the pump polarization on the emitted terahertz spectrum width. However, the central frequencies shift from 0.22 to 0.38 THz when the pump pulse polarization angle rotates 90°. When the polarization angle is 0°, it means the pump laser pulses are linearly polarized along with the optical axis of the lithium niobate crystal. For this case, the optical-to-terahertz energy conversion efficiency is maximized, and the effective interaction length is perfectly optimized, which is longer than that in the low-efficiency case. Since the linear absorption at higher terahertz frequencies is heavier than that at the lower frequencies, a longer effective interaction length implies that more of the higher terahertz frequencies will be absorbed when propagating inside the crystal. Hence, the emitted terahertz central frequency for the high-efficiency case is lower than that of the low-efficiency case, which agrees well with the experimental results.
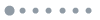
Figure 3.(a) Normalized output terahertz spectra for different rotation angles of the IR polarizer between the grating and the imaging lens. Zero degree means the pump laser is vertically polarized in the laboratory coordinate. (b) Normalized terahertz output spectra for the typical complementary angles, respectively. (c) Polarization angle dependence of the terahertz central frequency and spectral width. (d) Recorded terahertz signal as a function of the rotation angle of the IR polarizer.
Figure 4 shows the temporal waveforms before and after cutting in the knife-edge measurement. From this figure, we can see that the detected terahertz signal decreases along with moving the knife edge from the crystal angle of the emission plane. It is obvious that lots of terahertz energy is blocked by the metal knife, resulting in reduced terahertz signals detected by electro-optic sampling. From their corresponding Fourier transform spectra illustrated in Fig. 4(c), it can be seen that the recorded terahertz peak frequency shifts from a higher frequency of to a lower frequency of 0.17 THz when the knife blocks the emission signal generated at the positions close to the crystal cutting edge. Lithium niobate crystal has very strong linear absorption for terahertz waves, especially at room temperature. Our experiments are not conducted at low temperature. Therefore, from this aspect, the generated terahertz spectrum should have uniform frequency distribution when the knife edge is closer to the crystal cutting edge. When the knife edge was moved far away from the crystal phase matching angle, it is straightforward that higher frequency components would be more readily absorbed than lower frequencies due to a bigger absorption coefficient, leading to extracted lower-frequency components, which agrees very well with our obtained experimental results.
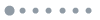
Figure 4.(a) Terahertz signal as a function of the knife-edge position. (b) Detected terahertz temporal waveforms in knife-edge measurement directly recorded after the crystal emission plane, and (c) their corresponding normalized Fourier transform spectra.
4. CONCLUSION AND OUTLOOK
Intense terahertz sources based on the tilted pulse front technique in lithium niobate are widely used in laboratories for terahertz nonlinear investigations. For mJ-level, super-intense terahertz sources, J-level pump laser sources are required. With a high-energy pump, nonlinear distortion is one of the non-negligible effects that may result in shifting of the generated terahertz beam position, varying its beam size, introducing spatial dispersion, and influencing the emission spectrum for the radiated terahertz waves. In this work, we experimentally investigate the terahertz emission spectrum influenced by the input infrared spectrum, pump polarization, and the emitted terahertz spatial dispersion in the intense terahertz generation process. We observe that the terahertz yield and emission spectrum are modulated not only by the temporal chirp added onto the pump pulses but also the input infrared spectrum. However, the emission spectrum does not rely on the infrared pump polarization, but its yield is slightly influenced by the rotation direction of the infrared polarizer. This provides us with an effective way to control the output terahertz energy for further application experiments. We also carefully characterize the terahertz emission spatial dispersion and find a slight spatial effect directly after the lithium niobate crystal. Our work not only helps to create deep understanding of the tilted pulse front theory but is also very useful for building intense terahertz sources and systems for further application experiments.
Acknowledgment
Acknowledgment. Dr. Xiaojun Wu thanks the Zhuoyue Program and Qingba Program of Beihang University.
References
[1] X. C. Zhang, A. Shkurinov, Y. Zhang. Extreme terahertz science. Nat. Photonics, 11, 16-18(2017).
[2] G. Q. Liao, Y. T. Li, Y. H. Zhang, H. Liu, X. L. Ge, S. Yang, W. Q. Wei, X. H. Yuan, Y. Q. Deng, B. J. Zhu, Z. Zhang, W. M. Wang, Z. M. Sheng, L. M. Chen, X. Lu, J. L. Ma, X. Wang, J. Zhang. Demonstration of coherent terahertz transition radiation from relativistic laser-solid interactions. Phys. Rev. Lett., 116, 205003(2016).
[3] H. A. Hafez, X. Chai, A. Ibrahim, S. Mondal, D. Ferachou, X. Ropagnol, T. Ozaki. Intense terahertz radiation and their applications. J. Opt., 18, 093004(2016).
[4] M. K. Liu, H. Y. Hwang, H. Tao, A. C. Strikwerda, K. B. Fan, G. R. Keiser, A. J. Sternbach, K. G. West, S. Kittiwatanakul, J. W. Lu, S. A. Wolf, F. G. Omenetto, X. Zhang, K. A. Nelson, R. D. Averitt. Terahertz-field-induced insulator-to-metal transition in vanadium dioxide metamaterial. Nature, 487, 345-348(2012).
[5] T. Kampfrath, K. Tanaka, K. A. Nelson. Resonant and nonresonant control over matter and light by intense terahertz transients. Nat. Photonics, 7, 680-690(2013).
[6] C. Lange, T. Maag, M. Hohenleutner, S. Baierl, O. Schubert, E. R. J. Edwards, D. Bougeard, G. Woltersdorf, R. Huber. Extremely nonperturbative nonlinearities in GaAs driven by atomically strong terahertz fields in gold metamaterials. Phys. Rev. Lett., 113, 227401(2014).
[7] O. Schubert, M. Hohenleutner, F. Langer, B. Urbanek, C. Lange, U. Huttner, D. Golde, T. Meier, M. Kira, S. W. Koch, R. Huber. Sub-cycle control of terahertz high-harmonic generation by dynamical Bloch oscillations. Nat. Photonics, 8, 119-123(2014).
[8] K. N. Egodapitiya, S. Li, R. R. Jones. Terahertz-induced field-free orientation of rotationally excited molecules. Phys. Rev. Lett., 112, 227401(2014).
[9] T. Maag, A. Bayer, S. Baierl, M. Hohenleutner, T. Korn, C. Schuller, D. Schuh, D. Bougeard, C. Lange, R. Huber, M. Mootz, J. E. Sipe, S. W. Koch, M. Kira. Coherent cyclotron motion beyond Kohn’s theorem. Nat. Phys., 12, 119-123(2016).
[10] M. A. W. Van Loon, N. Stavrias, N. H. Le, K. L. Litvinenko, P. T. Greenland, C. R. Pidgeon, K. Saeedi, B. Redlich, G. Aeppli, B. N. Murdin. Giant multiphoton absorption for THz resonances in silicon hydrogenic donors. Nat. Photonics, 12, 179-184(2018).
[11] C. Vicario, A. V. Ovchinnikov, S. I. Ashitkov, M. B. Agranat, V. E. Fortov, C. P. Hauri. Generation of 0.9-mJ THz pulses in DSTMS pumped by a Cr:Mg2SiO4 laser. Opt. Lett., 39, 6632-6635(2014).
[12] J. Hebling, G. Almasi, I. Z. Kozma, J. Kuhl. Velocity matching by pulse front tilting for large-area THz-pulse generation. Opt. Express, 10, 1161-1166(2002).
[13] X. J. Wu, J. L. Ma, B. L. Zhang, S. S. Chai, Z. J. Fang, C. Y. Xia, D. Y. Kong, J. G. Wang, H. Liu, C. Q. Zhu, X. Wang, C. J. Ruan, Y. T. Li. Highly efficient generation of 0.2 mJ terahertz pulses in lithium niobate at room temperature with sub-50 fs chirped Ti:sapphire laser pulses. Opt. Express, 26, 7107-7116(2018).
[14] H. Hirori, A. Doi, F. Blanchard, K. Tanaka. Single-cycle terahertz pulses with amplitudes exceeding 1 MV/cm generated by optical rectification in LiNbO3. Appl. Phys. Lett., 98, 091106(2011).
[15] S. W. Huang, E. Granados, W. R. Huang, K. H. Hong, L. E. Zapata, F. X. Kartner. High conversion efficiency, high energy terahertz pulses by optical rectification in cryogenically cooled lithium niobate. Opt. Lett., 38, 796-798(2013).
[16] J. A. Fulop, G. Polonyi, B. Monoszlai, G. Andriukaitis, T. Balciunas, A. Pugzlys, G. Arthur, A. Baltuska, J. Hebling. Highly efficient scalable monolithic semiconductor terahertz pulse source. Optica, 3, 1075-1078(2016).
[17] L. Palfalvi, G. Toth, L. Tokodi, Z. Marton, J. A. Fulop, G. Almasi, J. Hebling. Numerical investigation of a scalable setup for efficient terahertz generation using a segmented tilted-pulse-front excitation. Opt. Express, 25, 29560-29573(2017).
[18] B. K. Ofori-Okai, P. Sivarajah, W. R. Huang, K. A. Nelson. THz generation using a reflective stair-step echelon. Opt. Express, 24, 5057-5068(2016).
[19] L. Palfalvi, Z. Ollmann, L. Tokodi, J. Hebling. Hybrid tilted-pulse-front excitation scheme for efficient generation of high-energy terahertz pulses. Opt. Express, 24, 8156-8169(2016).
[20] S. C. Zhong, J. Li, Z. H. Zhai, L. G. Zhu, J. Li, P. W. Zhou, J. H. Zhao, Z. R. Li. Generation of 0.19-mJ THz pulses in LiNbO3 driven by 800-nm femtosecond laser. Opt. Express, 24, 14828-14835(2016).
[21] K. Ravi, W. R. Huang, S. Carbajo, E. A. Nanni, D. N. Schimpf, E. P. Ippen, F. X. Kartner. Theory of terahertz generation by optical rectification using tilted-pulse-fronts. Opt. Express, 23, 5253-5276(2015).
[22] C. Lombosi, G. Polonyi, M. Mechler, Z. Ollmann, J. Hebling, J. A. Fulop. Nonlinear distortion of intense THz beams. New J. Phys., 17, 083041(2015).
[23] F. Blanchard, X. Ropagnol, H. Hafez, H. Razavipour, M. Bolduc, R. Morandotti, T. Ozaki, D. G. Cooke. Effect of extreme pump pulse reshaping on intense terahertz emission in lithium niobate at multimilliJoule pump energies. Opt. Lett., 39, 4333-4336(2014).
[24] J. A. Fulop, Z. Ollmann, C. Lombosi, C. Skrobol, S. Klingebiel, L. Palfalvi, F. Krausz, S. Karsch, J. Hebling. Efficient generation of THz pulses with 0.4 mJ energy. Opt. Express, 22, 20155-20163(2014).
[25] M. Tsubouchi, K. Nagashima, F. Yoshida, Y. Ochi, M. Maruyama. Contact grating device with Fabry-Perot resonator for effective terahertz light generation. Opt. Lett., 39, 5439-5442(2014).
[26] M. I. Bakunov, S. B. Bodrov. Terahertz generation with tilted-front laser pulses in a contact-grating scheme. J. Opt. Soc. Am. B, 31, 2549-2557(2014).
[27] J. A. Fulop, L. Palfalvi, G. Almasi, J. Hebling. Design of high-energy terahertz sources based on optical rectification. Opt. Express, 18, 12311-12327(2010).
[28] J. A. Fulop, L. Palfalvi, S. Klingebiel, G. Almasi, F. Krausz, S. Karsch, J. Hebling. Generation of sub-mJ terahertz pulses by optical rectification. Opt. Lett., 37, 557-559(2012).
[29] S. B. Bodrov, A. A. Murzanev, Y. A. Sergeev, Y. A. Malkov, A. N. Stepanov. Terahertz generation by tilted-front laser pulses in weakly and strongly nonlinear regimes. Appl. Phys. Lett., 103, 251103(2013).
[30] M. Kunitski, M. Richter, M. D. Thomson, A. Vredenborg, J. Wu, T. Jahnke, M. Schoffler, H. Schmidt-Bocking, H. G. Roskos, R. Dorner. Optimization of single-cycle terahertz generation in LiNbO3 for sub-50 femtosecond pump pulses. Opt. Express, 21, 6826-6836(2013).
[31] X. J. Wu, S. Carbajo, K. Ravi, F. Ahr, G. Cirmi, Y. Zhou, O. D. Mucke, F. X. Kartner. Terahertz generation in lithium niobate driven by Ti:sapphire laser pulses and its limitations. Opt. Lett., 39, 5403-5406(2014).
[32] S. C. Zhong, Z. H. Zhai, J. Li, L. G. Zhu, J. Li, K. Meng, Q. Liu, L. H. Du, J. H. Zhao, Z. R. Li. Optimization of terahertz generation from LiNbO3 under intense laser excitation with the effect of three-photon absorption. Opt. Express, 23, 31313-31323(2015).
[33] L. Tokodi, J. Hebling, L. Palfalvi. Optimization of the tilted-pulse-front terahertz excitation setup containing telescope. J. Infrared Millimeter Terahertz Waves, 38, 22-32(2017).
[34] A. G. Stepanov, S. Henin, Y. Petit, L. Bonacina, J. Kasparian, J. P. Wolf. Mobile source of high-energy single-cycle terahertz pulses. Appl. Phys. B, 101, 11-14(2010).