Abstract
A wireless terahertz (THz) communication link is demonstrated, in which a THz quantum cascade laser and a THz quantum-well photo-detector (QWP) serve as the emitter and receiver, respectively. With the help of the well-matched THz QWP, the optical collection efficiency has greatly been improved. A data signal transmitted over 2.2 m with a low bit error rate () and data rate as high as 20 Mbps is achieved, which are almost 1 order of magnitude higher than that previously reported.Terahertz (THz; 0.1–10 THz) communications has received increasing interest in the field of wireless local area networks (WLANs) or wireless personal area networks (WPANs) due to the intrinsic advantages of potentially unrestricted frequency bands, ultrahigh bandwidth, and relatively secure channels[1,2]. Recently, several types of wireless communication links at THz frequency have been reported. These links employing uni-travelling carrier photodiode (UTC–PD) optoelectronics[3,4] and a resonant tunneling diode (RTD)[5] as the sources, mostly operating in the THz region between 0.3 and 0.6 THz. The higher frequency could facilitate a higher data rate. However, the development of THz wireless communication at high frequency is restricted by the intrinsic frequency of electronics-based devices.
Newly developed THz quantum photonic devices have exhibited great potential in high-frequency THz wireless communication, due to its advantages including fast intrinsic response speed, high sensitivity, and easy on-chip integration[6]. The quantum cascade laser (QCL) possesses unique high-frequency characteristics with theoretical band-widths in excess of 100 GHz[7]. The amplitude modulation of QCLs emitting in the 2–5 THz range up to 24 GHz has been already demonstrated[8], proving that THz QCLs have significant potential as high-speed data sources, particularly for free-space applications. Besides, a spectrally matched THz quantum-well photo-detector (QWP) is also a vital element in the high-frequency THz wireless communication[9–11]. Our group have previously reported a THz wireless communication system with a speed up to 5 Mbps via a system using a THz QCL as the emitter and a THz QWP as the receiver[12,13]. In this work, we designed a 3.27 THz wireless communication system based on a spectrally matched THz QWP. The wireless digital communication system achieved a data rate up to 20 Mbps with a low bit error rate (BER) value of less than .
The experimental setup is shown in Fig. 1. The receiver is a large-area THz QWP device with a size of . The detector with a peak at 3.2 THz, with a responsivity of about , is cooled down to 3.5 K by a continuous-flow liquid-helium cryostat. The emitter is a spectrally matched THz QCL, lasing at 3.27 THz, with an output power of about 6 mW in continuous wave mode by employing a hyper-hemispherical high-resistivity silicon lens customized by LongWave Photonics LLC. The laser is operated in a closed-cycle helium cryostat with the temperature of 11 K.
Sign up for Chinese Optics Letters TOC. Get the latest issue of Chinese Optics Letters delivered right to you!Sign up now
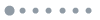
Figure 1.Scheme of the transmission setup based on a THz QCL and a THz QWP.
Normalized spectra of the QCL emission and the QWP response are illustrated in Fig. 2. For the THz QCL, the lasing frequency is 3.27 THz, which just locates in the responsive range of QWP (3–5 THz). As a receiver, the THz QCL is well-suited for improving the optical collection efficiency due to its high responsivity (94%) at 3.27 THz.
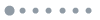
Figure 2.Normalized spectra of the THz laser emission and the detector response.
Moreover, the detector’s photocurrent was estimated using a low-noise current preamplifier (PA; SR570) directly connecting to the QWP. As it revealed in Fig. 3, when the output of the SR570 is 3.24 V, the value of detector photocurrent is 167 μA, almost 3 orders of magnitude higher than that in a previous report[14,15]. The great improvement of the detecting photocurrent is a result of the higher power of the QCL and the higher responsivity of the QWP, which enhanced and improved the SNR of the receiver greatly.
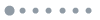
Figure 3.Measurement setup for the detector’s photocurrent based on a SR570.
The output signal of modulator circuit was controlled by the pseudorandom bit sequence (PRBS) signal from the BER analyzer, which led to a high and low-level switching. Here, the driven voltages of 12 and 14.9 V resulted in a low-level output signal (no radiation) and high-level output signal (radiation), respectively. As the electric field strength of the THz pulse directly depended on the driven voltages, the PRBS data were linearly mapped onto the THz wave and then formed the data’s envelop. Sufficient bandwidth of the modulator’s electrical path avoided inter-symbol interference (ISI), resulting in rectangular-shaped driving data pulses. Using two off-axis parabolic mirrors (PMs; PM1 and PM2) with a focal length of 101.6 mm, the emitting light from the QCL was transmitted through a 2.2 m optical path in free-space, and then focused onto the THz QWP mesa. Then the homemade signal processing circuit converted the current generated in the QWP to a voltage signal. The signal processing circuit was followed by a low-pass filter (LPF) with a 3 dB bandwidth of 20 MHz to eliminate the high-frequency excess noise caused by the detector and the signal processing circuit. Finally, the signal was fed into an oscilloscope and the BER analyzer’s decision gate.
For the first demonstration, a PRBS signal is transmitted at 20 Mbps over a 2.2 m distance based on a THz QCL and a well-matched THz QWP operated at 3.27 THz. A (PRBS)–9 () with an on-off keying–non-return-to-zero (OOK–NRZ) data stream is used to modulate the emitting source. This short-length random sequence is utilized as it is typically suitable for packet lengths in Ethernet networks. Figure 4 shows the measured (BER) performance against data rate of the system. The BER is rapidly increased with increasing bit rate, which can be attributed to the limited bandwidth of the signal processing circuit. Moreover, low BER () data transmission is achieved at a data rate as high as 20 Mbps and the eye diagram opens clearly. The data rates are almost 10 times as high as that in previously work[13]. Even the data rate speeds up to 22 Mbps, the related BER is , which could be reasonable for the application in WLANs.
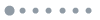
Figure 4.Measured BER performance versus data bit rate.
Eye diagrams of 18, 20, 22, and 24 Mbps are depicted in Figs. 5(a)–5(d), respectively. Although BERs over 20 Mbps, eye diagrams are still clearly open up. However, as the increasing of the data rate, the ISI becomes increasingly serious. Therefore, the increasing of the BER is caused by the ISI of received signal. The eye diagrams also reveal that the data-rate is dependent on the ISI, which is caused by limited receiver bandwidth.
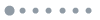
Figure 5.(a)–(d) Eye diagrams at different date rates.
The homemade signal processing circuit consists of a high gain trans-impedance amplifier (TIA) with bandwidth and a PA with a 3 dB bandwidth of 180 MHz. The SNR of the receiver achieves the maximum in condition of a photocurrent of around 167 μA and a high gain of the TIA, resulting in BERs below for the data rate up to 20 Mbps. The PA increases the voltage swing to at least 5 V before the transmission passes the LPF. For the bandwidth of the receiver components (PA and LPF) is higher than the measured data rate, the data rate is limited by the bandwidth of the TIA.
Due to the fact that the bandwidth of the TIA is inversely to the square root of the sum of the THz QWP capacitance and the capacitance of the cable in the cryostat from the cold section to room temperature, a higher data rate could be expected by utilizing the THz QWP and a cable with low capacitance. Moreover, the BER is determined by the received power and the background current of the THz QWP. Therefore, long-distance and error-free wireless links seem achievable using high-power THz QCLs and higher-response THz QWPs.
In conclusion, we achieve a 20 Mbps THz wireless communication link over a distance of 2.2 m employing a THz QCL as the source and a well-matched THz QWP as the receiver. The measured photocurrent of the THz QWP is about 167 μA, which is much enhanced and greatly improved the SNR of the receiver. Hence, eye diagrams are clearly open when the data rate up to 20 Mbps and BER lower than . A low BER transmission is obtained up to 20 Mbps, which is almost 1 order of magnitude higher than that in previously report[13]. Our further improvement will be focused on employing the THz QWP and a cable link with low capacitance, which will improve the bandwidth of TIA and the photocurrent of the THz QWP.
References
[1] T. Kleine-Ostmann, T. Nagatsuma. J. Infrared Millimeter Terahertz Waves, 32, 143(2011).
[2]
[3] T. Nagatsuma, S. Horiguchi, Y. Minamikata, Y. Yoshimizu, S. Hisatake, S. Kuwano, N. Yoshimoto, J. Terada, H. Takahashi. Opt. Express, 21, 23736(2013).
[4] S. Koenig, D. Lopez-Diaz, J. Antes, F. Boes, R. Henneberger, A. Leuther, A. Tessmann, R. Schmogrow, D. Hillerkuss, R. Palmer, T. Zwick, C. Koos, W. Freude, O. Ambacher, J. Leuthold, I. Kallfass. Nat. Photonics, 7, 977(2013).
[5] K. Ishigaki, M. Shiraishi, S. Suzuki, M. Asada, N. Nishiyama, S. Arai. Electron. Lett., 48, 582(2012).
[6] H. C. Liu, H. Luo, C. Y. Song, Z.-R. Wasilewski, A.-J. Spring–Thorpe, J. C. Cao. IEEE J. Sel. Top. Quantum Electron., 14, 374(2008).
[7] F. Capasso, R. Paiella, R. Martini, R. Colombelli, C. Gmachl, T. L. Myers, M. S. Taubman, R. M. Williams, C. G. Bethea, K. Unterrainer, H. Y. Hwang, D. L. Sivco, A. Y. Cho, A. M. Sergent, H. C. Liu, E. A. Whittaker. IEEE J. Quantum Electron., 38, 511(2002).
[8] W. Maineult, L. Ding, P. Gellie, P. Filloux, C. Sirtori, S. Barbieri, T. Akalin, J.-F. Lampin, I. Sagnes, H. E. Beere, D. A. Ritchie. Appl. Phys. Lett., 96, 021108(2010).
[9] H. C. Liu, C. Y. Song, A. J. Spring–Thorpe, J. C. Cao. Infrared Phys. Technol., 47, 169(2005).
[10] R. Zhang, X. G. Guo, C. Y. Song, M. Buchanan, Z. R. Wasilewski, J. C. Cao, H. C. Liu. IEEE Electron. Device Lett., 32, 659(2011).
[11] X. G. Guo, J. C. Cao, R. Zhang, Z. Y. Tan, H. C. Liu. IEEE J. Sel. Top. Quantum Electron., 19, 8500508(2013).
[12] Z. Chen, Y. J. Han, R. Zhang, X. G. Guo, H. Li, J. C. Cao, H. C. Liu. Electron. Lett., 47, 1002(2011).
[13] Z. Chen, L. Gu, Z. Tan, C. Wang, J. Cao. Chin. Opt. Lett., 11, 112001(2013).
[14] Z. Tan, Z. Chen, J. Cao, H. Liu. Chin. Opt. Lett., 11, 031403(2013).
[15] Q. Wu, L. Gu, Z. Tan, C. Wang, J. Cao. Chin. Opt. Lett., 12, 120401(2014).