Y. F. Chen1,*, C. C. Chang1, C. Y. Lee1, C. L. Sung1..., J. C. Tung1, K. W. Su1, H. C. Liang2, W. D. Chen3 and G. Zhang3|Show fewer author(s)
Abstract
The criterion of achieving efficient passive Q-switching is analyzed to design an off-axis pumped Nd:YVO4/Cr4+:YAG laser with a degenerate cavity. Experimental results reveal that pure high-order HG0,m or HGm,0 eigenmodes with the order m between 0 and 14 can be generated, depending on the off-axis displacement along the y axis or the x axis. On the other hand, lasing modes naturally turn into planar geometric modes when the off-axis displacement is larger than the value for exciting the HG0,m or HGm,0 eigenmodes with m>14. The overall peak powers for high-order eigenmodes or geometric modes can exceed 140 W. Furthermore, the high-order eigenmodes and geometric modes are employed to generate vortex beams with large orbital angular momentum by using an external cylindrical mode converter. Theoretical analyses are performed to confirm experimental results and to manifest the phase structures of the generated vortex beams.1. INTRODUCTION
Optical vortex beams have been exploited in a variety of applications, including optical tweezers and microscopy [1,2], particle trapping [3], controlling the chirality of twisted metal nanostructures [4,5], quantum communication [6,7], and spiral interferometry [8]. Even though the optical vortex beams can be obtained by directly generating the Laguerre–Gaussian (LG) modes with circular symmetry, it is generally difficult to make a laser oscillation in a pure LG mode. Alternatively, the optical vortex beams carrying orbital angular momentum (OAM) are generated by converting the high-order Hermite–Gaussian (HG) modes into LG modes via the use of an astigmatic mode converter (AMC) [9,10]. It has been verified that the HG modes can be directly generated in end-pumped solid-state lasers with selective pumping [11,12]. In addition to LG modes, the elliptical vortex beams can be similarly generated from the Ince–Gaussian (IG) modes—another form of eigenfunctions to the paraxial wave equation [13,14]. So far, most of the high-order HG and IG beams were generated in the continuous-wave (CW) operation with low output powers. Currently, generation of high-peak-power vortex beams with large OAM is an important and interesting topic for potential applications in quantum information, high-resolution optical trapping, and manipulating microparticles [15–17].
The -switched operation is a well-known method for generating high-peak-power lasers. Recently, passively -switched microchip lasers have been developed to generate various high-order transverse modes by means of adjusting the longitudinally focal position [18], the incident angle [19], or the polarization angle [20] of the pump beam. Even so, the transverse orders of lasing modes in microchip lasers usually increase with increasing the pump power due to the influence of thermal lens on the cavity configurations. It will be highly desirable to generate the high-peak-power vortex beams with large OAM and transverse order stability.
In this work, we analyze the criterion of the cavity length for achieving good passive Q-switching in an off-axis pumped laser with a concave-flat degenerate resonator. Based on the analysis, the cavity length is set to be the degenerate resonator with , where and are the transverse and longitudinal mode spacings, respectively. Under this circumstance, pure high-order or modes with order between 0 and 14 can be experimentally generated, depending on the off-axis displacement to be along the axis or the axis. On the other hand, the lasing modes turn out to be the planar geometric modes when the off-axis displacement is sufficiently large to excite orders higher than 15. The peak powers for both of high-order HG eigenmodes and planar geometric modes are overall greater than 140 W. Furthermore, the lasing modes are converted by an external AMC to generate the vortex beams with OAM. Experimental patterns of the converted beams are in good agreement with theoretical analyses. Based on the excellent agreement between theoretical analyses and experimental patterns, the phase structures of the transformed beams are numerically manifested.
2. ANALYSIS OF THE CAVITY DESIGN
Figure 1 illustrates the configuration of the concave-flat cavity for implementing the off-axis pumped passively -switched lasers in a degenerate resonator. Compared with Nd:YAG and Nd:YLF crystals for diode laser pumping, crystals possess the properties of large stimulated emission cross section at the lasing wavelength, high absorption coefficient, and wide absorption bandwidth at the pump wavelength. A larger stimulated emission cross section usually leads to a lower pumping threshold for the CW operation. Nevertheless, good passive -switching is subject to the second threshold condition [21] that the saturation in the absorber must occur before the gain saturation in the laser crystal. The coupled rate equations can be used to obtain the criterion for good passive -switching [22]: where is the ratio of the effective area in the gain medium to that in the saturable absorber, is the initial transmission of the saturable absorber, is the reflectivity of the output mirror, is the nonsaturable intracavity round-trip dissipative optical loss, is the ground-state absorption cross section of the saturable absorber, is the stimulated emission cross section of the gain medium, is the inversion reduction factor with a value between 0 and 2 as discussed in Ref. [23], and is the ratio of the excited-state absorption cross section to that of the ground-state absorption in the saturable absorber. By using a crystal as a saturable absorber in an laser, the typical values of the related parameters are given by [21], [22], , , , , and . Substituting these values into Eq. (1), it can be found that the ratio must be greater than 3.5 to achieve efficient passively -switching.
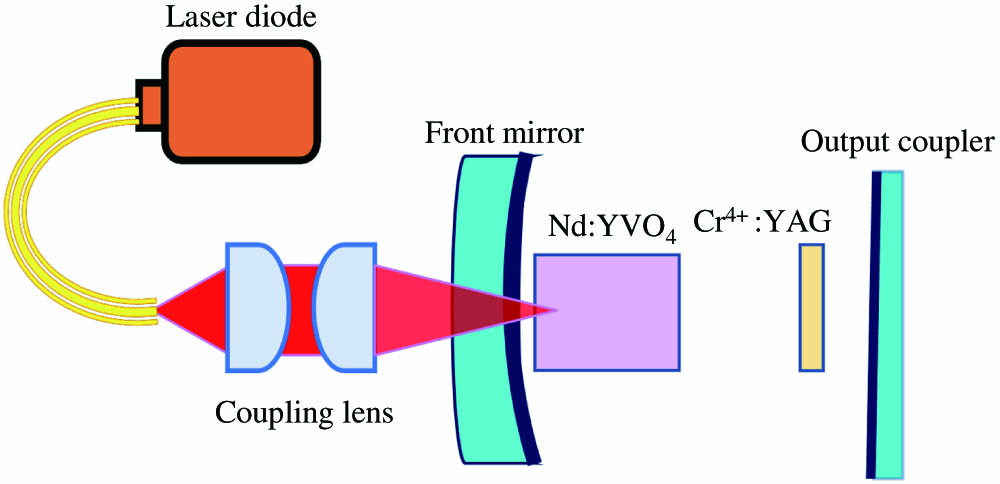
Figure 1.Configuration of the concave-flat cavity for implementing the off-axis pumped passively -switched lasers in a degenerate resonator.
For the cavity shown in Fig. 1, the mode sizes at the flat and concave mirrors are given by and [24], respectively, where is the emission wavelength, , is the radius of curvature of the concave mirror, is the effective cavity length given by , is the geometric length of the cavity, is the length of the laser crystal, is the length of the saturable absorber, is the refractive index of the laser crystal, and is the refractive index of the saturable absorber. Since the laser crystal and saturable absorber are very close to the concave and flat mirrors, respectively, the ratio can be estimated as for the first-order approximation. Using the criterion for good passive -switching, the criterion can be expressed as . Using , it can be derived that the cavity length must satisfy the criterion of to achieve good passive -switching.
On the other hand, the ratio between the transverse mode spacing and the longitudinal mode spacing for the present cavity is given by [25,26]. The condition for the degenerate cavity is expressed as , where and are co-prime integers. With simple algebra, the cavity lengths for the degenerate cavities can be given by . Using the criterion obtained in the last paragraph, it can be shown that the mode spacing ratio must satisfy the criterion of to achieve good passive -switching. In this work, the mode-spacing ratio is designed as to fulfill the criterion of good passive -switching. The cavity length for can be given by .
3. TRANSFORMING PLANAR GEOMETRIC MODES INTO CIRCULAR VORTEX BEAMS
The HG eigenmodes for the cavity with a concave mirror at and a plane mirror at are given by [25] where is the Gouy phase, is the Hermite polynomials of order , , is the eigenmode frequency, , and . The eigenmode frequency of the spherical cavity can be given by , where is the longitudinal mode index, and and are the transverse mode indices. It has been confirmed [11,12] that under the circumstance of the mode size matching , the high-order HG modes can be experimentally generated in the off-axis end-pumped solid-state lasers with and , where is the off-axis displacement of the pumping beam, is the radius of the pump beam, and is the average mode radius in the laser crystal. Consequently, the pure HG modes can be generated with the off-axis displacement and the mode size matching for the nondegenerate cavities.
Considering the effect of the degenerate cavity , the group of HG modes , with , can be found to constitute a family of frequency degenerate states, where and represent the minimum transverse and maximum longitudinal orders in the group, respectively. It has been experimentally found that the off-axis pumping with in the degenerate cavity can lead the emission mode to be the coherent superposition of the degenerate states if the displacement is greater than the critical value. In the following section, the critical displacement is experimentally found to be approximately . Note that all the discussion for the off-axis pumping with can be applied to the case of for the group of the degenerate states . It has been experimentally confirmed that the emission mode can be characterized with the SU(2) coherent state [26,27] where stands for the number of HG modes. The wave patterns of the coherent states are verified to manifest the ray–wave correspondence, and the phase factor can be used to link to the starting ray of the geometric periodic orbits [26–31]. Figure 2 shows the wave patterns inside the cavity for the cases of and with and . The wave patterns inside the cavity can be confirmed to exactly correspond to the geometric ray orbits. As a result, the coherent states are called the geometric modes. The transverse patterns of the emitted beams at are shown on the right-hand side of Fig. 2. The lasing modes can be interpreted as the multiple Gaussian beams distributed in the direction.
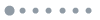
Figure 2.Wave patterns inside the cavity for the cases of (a) and (b) with and . Right-hand side: transverse patterns of the emitted beams at .
With an external -cylindrical-lens mode converter [9,10], HG modes can be transformed into LG modes to generate the vortex beam with OAM. Consequently, the transformed beam of the planar geometric mode is given by where are the associated Laguerre polynomials and is the lasing wave number. The sign of in Eq. (5) depends on the angle of the mode converter with respect to the axis. The phase structure of the converted beam is given by
Figure 3 depicts numerical results of the wave patterns with a fixed for three cases of . The second row in Fig. 3 shows numerical results for the phase structures . It can be seen that the charge of the central vortex is determined by the value of . In addition, the central vortex is surrounded by the singularities with charges of , the total number of which is equal to .
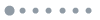
Figure 3.Numerical results for the wave patterns with a fixed for three cases of , 10, and 15. Second row: numerical results for the phase structures.
4. EXPERIMENTAL RESULTS AND DISCUSSION
The concave input mirror had an antireflection coating at 808 nm (reflection ) on the entrance face, a high-reflectance coating at 1064 nm (reflection ), and a high transmittance coating at 808 nm (transmission ) on the second surface. The gain medium was -cut 0.2 at. % crystal with a length of 12 mm and placed very near (1–2 mm) the input mirror. The flat output coupler had a 5% transmission coating at 1064 nm on the first surface and an antireflection coating at 1064 nm (reflection ) on the second surface. A crystal with an initial transmission of at 1064 nm was used as a saturable absorber and was placed very near (1–2 mm) the output coupler. Both end surfaces of the laser crystal and saturable absorber were coated for antireflection at 1064 nm (reflection ). Moreover, both the laser crystal and saturable absorber were wrapped with indium foil and mounted in water-cooled copper holders with a temperature of 20°C. The pump source was a 10 W 808 nm fiber-coupled laser diode with a core diameter of 100 μm and a numerical aperture of 0.16. The average pump diameter was approximately 130 μm. Since the radius of curvature of the concave mirror is , the effective cavity length for can be calculated to be approximately 27.14 mm. Considering the lengths of the laser crystal and saturable absorber, the geometric length of the laser cavity is set to be approximately 34.0 mm.
The pumping beam was initially aligned at the transverse center of the laser cavity to excite the fundamental Gaussian mode. Subsequently, the pumping beam was displaced in the direction to generate high-order modes. It was experimentally found that the lasing mode was the HG mode for and the planar geometric mode for . The order for the HG modes was approximately given by , where was estimated to be approximately 0.13 mm by considering the thickness of the laser crystal. Figure 4(a) shows the average output power versus the incident pump power for the lasing modes excited at , 0.55, and 0.97 mm. The dependence of the lasing mode on the pump power is shown in Fig. 4(b), where the transverse patterns were measured at . Experimental transverse patterns can be seen to be fairly sustained for pump powers below 3.8 W. By using Eqs. (3) and (4) to analyze experimental patterns, the lasing modes excited at , 0.55, and 0.97 mm are found to be , , and , respectively. At a pump power of 3.8 W, the average output powers are 0.78, 0.67, and 0.31 W for , , and modes, respectively.
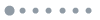
Figure 4.(a) Average output power versus incident pump power for lasing modes excited at , 0.55, and 0.97 mm. (b) Dependence of the lasing mode on the pump power.
Figure 5(a) shows the pulse repetition rate versus the incident pump power for the lasing modes shown in Fig. 4(a). At a pump power of 3.8 W, the pulse repetition rates can be up to 128, 134, and 103 kHz for , , and modes, respectively. By using the average output powers shown in Fig. 4(a), the pulse energies can be calculated to be approximately 6.1, 5.0, and 3.0 μJ, for , , and modes, respectively. A typical oscilloscope trace is presented in Fig. 5(b). The overall pulse-to-pulse amplitude fluctuation of the -switched pulse train was found to be less than . The widths of single pulses for , , and modes were measured to be 30, 26, and 22 ns, respectively. The peak power can be generally higher than 140 W. The higher peak power is expected to be obtained by using a crystal with lower initial transmission. High-peak-power vortex beams can be useful in wavelength conversion and material processing.
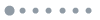
Figure 5.(a) Pulse repetition rate versus incident pump power for lasing modes shown in Fig. 4(a). (b) A typical oscilloscope trace.
The AMC formed by a matched pair cylindrical lenses is one of the well-known methods to generate optical vortex beams via converting HG into LG [9,10]. With an external -cylindrical-lens mode converter, the planar geometric modes can be transformed into circularly geometric modes when the axes of the cylindrical lenses are parallel to one another but at to the principal axes of the planar geometric modes. The focal length of the cylindrical lenses is ; the distance is precisely adjusted to be for the operation of the converter. Figure 6 shows experimental results (first row) and numerical calculations (second row) for the patterns of the converted beams at for the lasing modes shown in Fig. 4(b) at a pump power of 3.8 W. Numerical calculations are in good agreement with experimental observations for all cases. The third row in Fig. 6 shows numerical results for the phase structures.
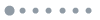
Figure 6.Experimental results (first row), numerical calculations (second row), and numerical phase structures (third row) for the patterns of the converted beams at for the lasing modes shown in Fig. 4(b) at a pump power of 3.8 W.
5. CONCLUSIONS
In summary, we have analyzed the criterion of achieving good passive -switching to design an off-axis pumped laser with a degenerate cavity of . It is experimentally found that pure high-order or modes with the order between 0 and 14 can be generated, depending on the off-axis displacement to be along the axis or the axis. When the off-axis displacement is sufficiently large enough to excite the transverse order higher than 15, the lasing modes are found to naturally turn into planar geometric modes. On the whole, the peak powers for high-order eigenmodes or geometric modes can exceed 140 W. To generate vortex beams with large OAM, high-order eigenmodes and geometric modes are transformed by an external AMC. Theoretical analyses have also been performed to confirm experimental results and to manifest the phase structures of the transformed beams.
References
[1] M. Woerdemann, C. Alpmann, M. Esseling, C. Denz. Advanced optical trapping by complex beam shaping. Laser Photon. Rev., 7, 839-854(2013).
[2] V. G. Shvedov, A. V. Rode, Y. V. Izdebskaya, A. S. Desyatnikov, W. Krolikowski, Y. S. Kivshar. Giant optical manipulation. Phys. Rev. Lett., 105, 118103(2010).
[3] K. T. Gahagan, G. A. Swartzlander. Optical vortex trapping of particles. Opt. Lett., 21, 827-829(1996).
[4] K. Toyoda, K. Miyamoto, N. Aoki, R. Morita, T. Omatsu. Using optical vortex to control the chirality of twisted metal nanostructures. Nano Lett., 12, 3645-3649(2012).
[5] K. Toyoda, F. Takahashi, S. Takizawa, Y. Tokizane, K. Miyamoto, R. Morita, T. Omatsu. Transfer of light helicity to nanostructures. Phys. Rev. Lett., 110, 143603(2013).
[6] G. Molina-Terriza, J. P. Torres, L. Torner. Twisted photons. Nat. Phys., 3, 305-310(2007).
[7] G. Gibson, J. Courtial, M. J. Padgett, M. Vasnetsov, V. Pas’ko, S. M. Barnett, S. Franke-Arnold. Free-space information transfer using light beams carrying orbital angular momentum. Opt. Express, 12, 5448-5456(2004).
[8] A. Jesacher, S. Fürhapter, S. Bernet, M. R. Marte. Spiral interferogram analysis. J. Opt. Soc. Am. A, 23, 1400-1409(2006).
[9] M. W. Beijersbergen, L. Allen, H. E. L. O. van der Veen, J. P. Woerdman. Astigmatic laser mode converters and transfer of orbital angular momentum. Opt. Commun., 96, 123-132(1993).
[10] E. G. Abramochkin, V. G. Volostnikov. Beam transformations and nontransformed beams. Opt. Commun., 83, 123-135(1991).
[11] Y. F. Chen, T. M. Huang, C. F. Kao, C. L. Wang, S. C. Wang. Generation of Hermite–Gaussian modes in fiber-coupled laser-diode end-pumped lasers. IEEE J. Quantum Electron., 33, 1025-1031(1997).
[12] H. Laabs, B. Ozygus. Excitation of Hermite–Gaussian modes in end-pumped solid-state lasers via off-axis pumping. Opt. Laser Technol., 28, 213-214(1996).
[13] M. A. Bandres, J. C. Gutiérrez-Vega. Ince–Gaussian modes of the paraxial wave equation and stable resonators. J. Opt. Soc. Am. A, 21, 873-880(2004).
[14] T. Ohtomo, K. Kamikariya, K. Otsuka, S. Chu. Single-frequency Ince–Gaussian mode operations of laser-diode-pumped microchip solid-state lasers. Opt. Express, 15, 10705-10717(2007).
[15] J. Hamazaki, R. Morita, Y. Kobayashi, S. Tanda, T. Omatsu. Laser ablation using a nanosecond optical vortex pulse. Proceedings of IEEE Conference on CLEO/Europe-EQEC, CC1.5 THU(2009).
[16] G. B. Jung, K. Kanaya, T. Omatsu. Highly efficient phase-conjugation of a 1 μm pico-second Laguerre–Gaussian beam. Opt. Express, 14, 2250-2255(2006).
[17] Y. Tanaka, M. Okida, K. Miyamoto, T. Omatsu. High power picosecond vortex laser based on a large-mode-area fiber amplifier. Opt. Express, 17, 14362-14366(2009).
[18] J. Dong, S. C. Bai, S. H. Liu, K. I. Ueda, A. A. Kaminskii. A high repetition rate passively Q-switched microchip laser for controllable transverse laser modes. J. Opt., 18, 055205(2016).
[19] J. Dong, Y. He, S. C. Bai, K. I. Ueda, A. A. Kaminskii. A Cr4+:YAG passively Q-switched Nd:YVO4 microchip laser for controllable high-order Hermite–Gaussian modes. Laser Phys., 26, 095004(2016).
[20] H. S. He, M. M. Zhang, J. Dong, K. I. Ueda. Linearly polarized pumped passively Q-switched Nd:YVO4 microchip laser for Ince–Gaussian laser modes with controllable orientations. J. Opt., 18, 055205(2016).
[21] Y. F. Chen, S. W. Tsai. Simultaneous Q-switching and mode-locking in a diode-pumped Nd:YVO4–Cr4+:YAG laser. IEEE J. Quantum Electron., 37, 580-586(2001).
[22] G. Xiao, M. Bass. A generalized model for passively Q-switched lasers including excited state absorption in the saturable absorber. IEEE J. Quantum Electron., 33, 41-44(1997).
[23] J. J. Degnan, D. B. Coyle, R. B. Kay. Effects of thermalization on Q-switched laser properties. IEEE J. Quantum Electron., 34, 887-899(1998).
[24] N. Hodgson, H. Weber. Laser Resonators and Beam Propagation(2005).
[25] A. E. Siegman. Lasers(1986).
[26] Y. F. Chen, C. H. Jiang, Y. P. Lan, K. F. Huang. Wave representation of geometrical laser beam trajectories in a hemiconfocal cavity. Phys. Rev. A, 69, 053807(2004).
[27] Y. F. Chen, J. C. Tung, P. Y. Chiang, H. C. Liang, K. F. Huang. Exploring the effect of fractional degeneracy and the emergence of ray–wave duality in solid-state lasers with off-axis pumping. Phys. Rev. A, 88, 013827(2013).
[28] J. Erhard, H. Laabs, B. Ozygus, H. Weber. Diode-pumped multipath laser oscillators. Proc. SPIE, 3611, 2-10(1999).
[29] Q. Zhang, B. Ozygus, H. Weber. Degeneration effects in laser cavities. Eur. Phys. J. Appl. Phys., 6, 293-298(1999).
[30] J. Dingjan, M. P. van Exter, J. P. Woerdman. Geometric modes in a single-frequency Nd:YVO4 laser. Opt. Commun., 188, 345-351(2001).
[31] A. A. Malyutin. Closed laser-beam trajectories in plano-spherical resonators with Gaussian apertures. Quantum Electron., 38, 181-186(2008).