Abstract
The mid-infrared (mid-IR) second-order optical nonlinearity of the barium titanate (BTO) thin films was characterized by second harmonic generation (SHG). The epitaxial BTO thin films were grown on strontium titanate substrates by pulsed-laser deposition. From the azimuthal-dependent polarized SHG measurements, the tensorial optical nonlinear coefficients, , and ferroelectric domain fraction ratio, , were resolved. Strong SHG signals were obtained at the pumping laser wavelength between 3.0 and 3.6 μm. The SHG intensity was linearly dependent upon the square of the pumping laser power. The broadband mid-IR optical nonlinearity enables BTO thin films for applications in chip-scale quantum optics and nonlinear integrated photonic circuits.1. INTRODUCTION
Perovskite oxides, such as , , and , are attractive materials because they exhibit excellent ferroelectric and nonlinear optical properties at room temperature. Their ferroelectric properties lead to a variety of applications including sensors [1], non-volatile memory devices [2], and optical communications [3,4]. Perovskite oxides have the chemical formula , where their polar property determines the polarization, which is the net dipole moment in the unit volume. The nonlinear interactions between the electric dipole and light waves create nonlinear optical responses such as SHG, sum-frequency generation (SFG), difference-frequency generation (DFG), and optical parametric oscillation (OPO) [5]. One application for nonlinear optic technology is to provide light sources over ultraviolet, visible, infrared, and THz bands through nonlinear light conversion [6–8]. Another critical application for nonlinear optic technology includes quantum optics, where entangled photon pairs are efficiently created during the nonlinear optical processes [9–11].
Though bulk crystalline perovskite oxides have a strong optical nonlinearity, a thin film form is required to monolithically integrate these perovskites with photonic circuits. Perovskite oxide thin films can be grown by numerous epitaxy techniques such as metal oxide chemical vapor deposition (MOCVD), molecule-beam epitaxy (MBE), and pulsed-laser deposition (PLD) [12–14]. Among these perovskite oxide thin films, BTO has attracted much attention and been utilized in various micro- and nano-photonic devices for the following reason [15–18]: BTO thin films have a broad transparent window in visible and IR range [19,20]. Through the Pockels effect, the refractive indexes of BTO thin films are tunable by applying external electric fields [21,22]. The second-order nonlinear susceptibilities of BTO can be further enhanced by inducing stress in the BTO thin film [23]. Moreover, BTO thin films can be epitaxially grown on the Si substrates with an intermediate buffer layer [24,25], thus providing additional functionality for present Si photonic devices.
The optical nonlinearity of BTO thin films has been explored in the visible and near-IR regions [26–28], while not in the mid-IR region, which is an important spectrum for the development of optical networking [29], biomedical sensing [30], molecular spectroscopy [31], and thermal imaging [32]. For optical communication, a broader spectrum extending into the mid-IR region can accommodate additional channels for high-speed data transmission. Moreover, mid-IR sensing enables label-free and non-destructive molecule detection [33,34]. In this study, the epitaxial BTO thin films were deposited onto a strontium titanate (STO) substrate by PLD. Polarization-dependent mid-IR SHG was measured and analyzed to resolve the optical nonlinear coefficients and the ferroelectric domain distribution. Our results illustrated that the epitaxial BTO thin film is an ideal platform for mid-IR nonlinear integrated photonics.
Sign up for Photonics Research TOC. Get the latest issue of Photonics Research delivered right to you!Sign up now
2. EPITAXIAL BTO THIN FILM GROWTH AND XRD CHARACTERIZATION
The BTO thin film was deposited onto a single-crystal STO (001) substrate by PLD at 600°C and 20 mTorr of . The PLD used a KrF excimer laser () with a fluence of and a repetition rate of 5 Hz. The BTO target was prepared by a conventional ceramic sintering method. The grown BTO film was 500-nm thick with a uniform surface. The crystalline structure of the BTO thin film was characterized by X-ray diffraction (XRD). From the scan in Fig. 1(a), only BTO () was found, indicating that the BTO thin film is a single crystalline film and mainly grown along the () direction. The scans of BTO (113) and STO (113) in Fig. 1(b) indicate that the BTO thin film exhibited cube-on-cube growth on the STO substrate without experiencing in-plane rotations.
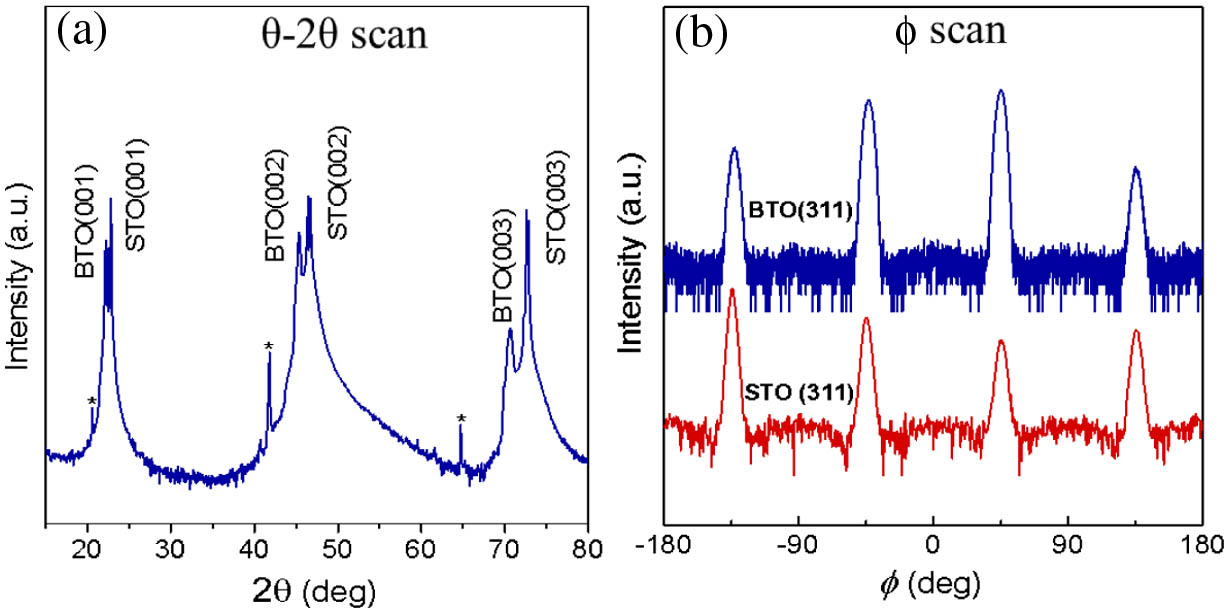
Figure 1.XRD results of the BTO thin film deposited on the (001) STO substrate by PLD. (a) A scan of the BTO thin film. The BTO was epitaxially grown along the () direction. (b) A scan of the BTO thin film indicated a cube-on-cube growth on the STO substrate.
3. MODELING OF THE MID-IR SHG FROM BTO THIN FILMS
A BTO crystal possesses different crystal symmetries at different temperatures due to its phase transitions. Upon increasing the temperature, the phase of the BTO crystal changes sequentially from rhombohedral to orthorhombic, tetragonal (at 0–120°C), and paraelectric cubic phase. The space group of tetragonal BTO is P4mm and its unit cell is slightly elongated along the c-direction, which is the fourfold axis as shown in Fig. 2(a). The distance from the center Ti atom to the O atoms along the c-axis can be greater or smaller than to the other O atoms along the a-axis, leading to a spontaneous polarization parallel to the c-axis [35]. For an epitaxial BTO thin film, the coordinate axes were defined by the crystal axes of the STO substrate. As shown in Fig. 2(b), the [001], [100], and [010] axes of the STO are defined as the , , and axes, respectively. A BTO thin film can have six different ferroelectric domain variants with their polarizations parallel to the , , , , , and directions, which are labeled as the , , , , , and domains, respectively. To study the polarimetric SHG, Fig. 3 illustrates that the , , and axes of the lab coordination are initially aligned to the , , and axes defined by the crystal coordination of the STO substrate [36]. In other words, a light beam propagating along the -axis is normally incident to the (001) surface of the STO substrate, and its polarization is in the plane. At azimuth , the polarization direction is coincident with the -axis. To analyze the polarimetric SHG from a multi-domain BTO thin film, a polarizer was placed before the photodetector to differentiate the and polarized SHG signals, where the intensities are denoted as and , respectively. For propagating in the -direction, the and domain variants will not create an SHG polarization in the plane.
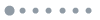
Figure 2.(a) Structure of a tetragonal BTO unit cell with one Ti atom in the cell center, eight Ba atoms in the corners, and six O atoms in the center of the facets. (b) The six possible ferroelectric domain variants, , , , , , and , in the , , and lab-based coordinate system.
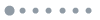
Figure 3.Four possible domain variants in the plane, , , , and , and the crystal axes (, , ) associated with each of the four domain variants. The incident light is linearly polarized, and the azimuthal angle between the incident light and the -axis is noted as .
The SHG collected from a multi-domain BTO thin film was considered as a combination of the SHG signals attributed by each domain variant. To use the nonlinear optical tensor matrix, the components of the incident electric field (, , ) were transformed and interpreted by the orthogonal crystal coordination (, , ) corresponding to each domain variant. Here, the optic axis of the crystal is parallel to the direction of and equivalent to the c-axis indicated in Fig. 2(a).
Figure 3 displays the new coordinations (, , ) and the direction associated with the , , , and domain variants, separately. The second-order nonlinear polarization for the tetragonal BTO is then described as , , and are the components of the electrical fields along the , , and axes, and is the tensor element of the second-order optical nonlinear coefficients. The electric field of the light propagating along the x-direction has , , and . Considering the domain, the electric fields represented by the domain coordination became , , and . Hence, by applying this to Eq. (1), the nonlinear polarizations from the domain are expressed as In addition, the SHG intensity, , is proportional to , and for the domain, and are parallel to and , respectively. Therefore, it follows that The reported SHG coefficients of bulk BTO crystal at are , , and [23]. Figure 4 plots the and polarized SHG signals created from the domain when the incident light polarization was rotated along on the plane. exhibited a two-lobed intensity profile, while had a distinguishable four-lobed profile. Similarly, the nonlinear polarizations contributed by the , , , and domains were calculated and represented by the domain coordinates, which are summarized as
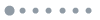
Figure 4.Calculated azimuthal-dependent polarized SHG, and , of a single domain BTO thin film. The tensors from a bulk BTO crystal were used: , , and . The thickness of the BTO crystal was 500 nm. and showed distinct two-lobed and four-lobed SHG patterns, respectively.
When the pump light passed through a BTO thin film containing different ferroelectric domains, the SHG contributed by each of these domains needs to be considered. The area fractions of each domain are defined as , , , and , where the sum of all the area fractions is 1. From the transformation between the domain variants and the lab coordinates shown in Fig. 2, it was concluded that , , , and . Using Eqs. (7)–(10), the total nonlinear polarization created by the four domains, , , , and , is and are defined as and , which present the difference in the area fractions between the domain pairs, such as (, ) and (, ), oriented along the opposite directions. From Eq. (11), is strongly correlated with the domain orientation and the domain fraction of the opposite polarization. Due to the difference of the refractive indexes along the c- and a-axes of the BTO, a phase term was added to Eq. (11) to account for the birefringence between the c- and a-axes. Here, is the thickness of the thin film sample, and and are the refractive indexes at the second harmonic frequency along the c- and a-axes of BTO, respectively. If these four domains have equal areas, no SHG signal will be observed because and become zero in Eq. (11). Since , the SHG intensity of the -polarization is For convenience, the value of was set to 1. The expression of -polarized SHG is then rewritten as where , , , and .
Using the same approach, the intensity of the -polarized SHG is where , , , and . The parameters derived from the and polarizations have the following relations: and The SHG intensity can be calculated by Eqs. (13) and (14) when all the are known. On the other hand, and the associated material parameters, including the domain fraction difference and nonlinear coefficient , can be extrapolated by fitting the experimentally obtained SHG. In parallel, Eqs. (15) and (16) establish the relationship between the eight identities and validate the model used to interpret the domain orientation and the optical nonlinearity.
Hence, the polarimetric response of the SHG is determined by the domain fraction ratio and the optical nonlinearity . To examine the effect of , Figs. 5(a)–5(c) show the calculated angular-dependent and by applying Eqs. (13) and (14) at , 1, and 0.1, respectively. The values of the optical nonlinear coefficients, , , and , were obtained from a bulk BTO crystal. As shown in Fig. 5(a), at , the domains were the dominant factor for the polarimetric SHG pattern, resulting in a two-lobed profile and a four-lobed profile. The two-lobed had its maximum SHG intensity at and 260°. On the other hand, the four-lobed had its maximum SHG intensity at and 235°, and its other two peaks had maximum SHG intensities at and 315°. The SHG patterns at were similar to the SHG patterns in Fig. 4, which were created from a single domain BTO, except that the two lobes of the were slightly tilted away from the vertical axis, and the maximum SHG intensities from those four lobes in were different. When was decreased to 1, the and domains had comparable contributions to the SHG, thus creating and patterns with similar profiles as shown in Fig. 5(b). Both and have two lobes, and these lobes are approximately 45° away from the horizontal axis. Once was further decreased to 0.1, the domains became the dominant factor for the polarimetric SHG pattern. As a result, a four-lobed profile and a two-lobed profile were found and are illustrated in Fig. 5(c). Unlike Fig. 5(a) where the two lobes from were closely aligned to the vertical axis, the other two lobes from in Fig. 5(c) were almost aligned with the horizontal axis. Therefore, the symmetry and the angular dependence of the polarimetric SHG patterns are highly dependent on the domain fraction ratio. In other words, can be resolved by examining the variation of the profiles.
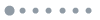
Figure 5. and were calculated when the ferroelectric domain fraction ratio, , was set to (a) 10, (b) 1, and (c) 0.1. The values were from a bulk BTO crystal. At , had a two-lobed profile, and had a four-lobed profile. As decreased to 0.1, became four-lobed and became two-lobed. In addition, the axis of the two-lobes rotated as changed.
4. POLARIMETRIC MID-IR SHG MEASUREMENTS
A. Experimental Setup
Figure 6 shows the schematic of the experimental setup for measuring the polarimetric SHG from a BTO thin film. A wavelength-tunable OPO pulsed laser was used as the mid-IR light source. The pulse repetition rate was 1 kHz, and the linewidth was smaller than . A bandpass filter with a transmission window from to 3.75 μm was placed after the laser. The maximum transmission was 80% at . This filter was used to block the light frequencies other than the laser beam that had a pumping frequency of . After the first bandpass filter, the laser beam was focused onto the sample surface through a mid-IR objective lens to maximize the incident light intensity. The focusing length and the numerical aperture of the mid-IR objective lens were 6 mm and 0.25, respectively. The incident laser beam was projected along the -direction. By rotating the half-wave plate, the polarization of the laser beam was also rotated by an azimuth angle along the -axis. The half-wave plate had a 100% transmission and 1.5 wave retardance at . The BTO thin film sample was initially placed on a rotating stage to align the sample axes with the lab axes, so that , , and . Otherwise, there will be an angle between the principle c-axis of the sample and the lab coordinate -axis. The angle between the lab coordinate -axis and the direction normal to the BTO thin film surface is so that the input light was normally incident to the sample. To effectively collect all of the SHG signal, the light emitted from the BTO sample was collected and then refocused into a detector by two separate objective lenses. An ultra-broadband wire-grid polarizer operational at was placed after the objective lens as an analyzer to differentiate the polarized SHG, or . The extinction ratio of the wire-grid polarizer was . A second bandpass filter with a transmission window from to 2.0 μm was placed in front of the detector to remove the remaining incident laser beam at frequency . Thus, only the generated SHG signal at was collected. The detector was a PbSe fixed gain photodetector sensitive from to 4.8 μm with a bandwidth range from 0.2 Hz to 10 kHz. The detector was connected to an oscilloscope, and the SHG signal at various was recorded from the amplitude of the pulse displayed on the oscilloscope.
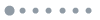
Figure 6.Schematic of the experimental setup for measuring the azimuthal-dependent polarized SHG from a BTO thin film. The pumping laser was a tunable ns pulsed laser and the first filter removed the light that was not at the pumping wavelength . The polarization of the light was rotated by the phase plate. The mid-IR light was focused on the BTO thin film using the front objective lens, and the SHG was collected by another objective lens in the back. The polarizer selected the polarization of the SHG signals, and the second filter removed the residual mid-IR pumping light. The SHG in the NIR region was measured by a photodetector or a spectrometer.
B. Mid-IR Second-Order Optical Nonlinearity and Ferroelectric Domain Fractions
To investigate the optical nonlinearity and the ferroelectric domains of the BTO thin film, the azimuthal-dependent SHG signals along the and polarizations, and , were measured and displayed in Figs. 7(a) and 7(b), respectively. The substrate STO had no second-order nonlinearity due to its crystal symmetry. Both and polarized SHG patterns show a dominant two-lobed profile with a pair of smaller lobes. For , the maximum SHG signal was found at a relative and 135°. The smaller lobes were found to be normal to the larger lobes. Similar profiles were observed for , where a pair of larger lobes and another pair of secondary lobes were found. A minor difference between the and is that the intensities of the secondary lobes were comparatively weaker in than in . The SHG profiles shown in Fig. 7 were fitted by Eqs. (13) and (14). Accordingly, , , , and were obtained. The fitting results were consistent with the observation shown in Fig. 4, where and revealed a two-lobed dominant pattern when approached 1. In addition, the difference in between a bulk BTO crystal and the BTO thin film is due to the film strain introduced during PLD growth. A similar effect has been observed in studies using other perovskite oxides including and [37].
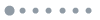
Figure 7.(a) and (b) obtained from the BTO thin film deposited by PLD. The dashed lines represent the measured data and the solid green lines represent the modeling results. From the fitting, the values , , , and were resolved.
C. Mid-IR SHG Dependence on the Pumping Light Intensity
The variation of the SHG at different pumping light intensities was investigated. During the measurement, the laser power was gradually increased from 16 to 92 mW. The laser wavelength was and the azimuth angle was fixed at 45° because reached its maximum at 45° according to Fig. 7(a). Figure 8 displays the SHG intensity versus the square of the pumping light intensity, , where was measured by a digital optical power meter (Thorlabs PM100D). The SHG intensity increased proportionally to when gradually increased from 16 to 92 mW. The mid-IR SHG pump threshold was 7 mW. The linear dependence obtained between and agrees with the derivation illustrated in Eqs. (5) and (6).
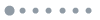
Figure 8.SHG intensity, , versus the square of the input mid-IR laser power, , measured at . The black circles indicate the measured data, and the red line represents the fitted curve. A linear relationship between and was found.
D. Mid-IR SHG Spectral Characterization
The SHG spectrum from the BTO thin film was measured when the wavelength of the pumping laser was tuned over a range between and 3.6 μm. The SHG signal was collected by a 400 μm core diameter fiber and analyzed by a spectrometer that had a thermoelectric cooling InGaAs linear array. The azimuthal angle and the position of the BTO sample were fixed when the input wavelength was tuned. As drawn in Fig. 9, the center wavelength of the SHG spectrum increased from to 1.8 μm when the laser pumping wavelength increased from to 3.6 μm. The slight broadening of the SHG spectrum at was due to variation in the pumping laser linewidth. The spectral scanning of the SHG signals demonstrates that the deposited BTO thin film can convert mid-IR signals over a wide spectrum efficiently into the NIR region, thus enabling on-chip and broadband nonlinear frequency conversion.
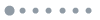
Figure 9.SHG spectrum of the BTO thin film when the mid-IR pumping wavelength was tuned from to 3.6 μm. Strong SHG signals between and 1.8 μm were found, indicating that BTO has a broadband second-order optical nonlinearity.
5. CONCLUSIONS
Broadband mid-IR SHG was demonstrated by an epitaxial BTO thin film on a (001) STO substrate using PLD. From XRD characterization, the BTO film had cube-on-cube growth mainly along the (00l) direction. The mid-IR optical nonlinearity and the ferroelectric domain property were characterized by azimuthal-dependent polarized SHG measurements. Two-lobed dominant patterns were found in both polarizations. High nonlinear coefficients of , , and , and a uniform domain fraction ratio of were resolved. In addition, the characteristic SHG response, , and broadband SHG signals were observed at pumping wavelengths –. The efficient nonlinear thin film paves the way for developing efficient on-chip light sources and integrated quantum photonics.
Acknowledgment
Acknowledgment. The characterization of the devices was accomplished at the Mid-Infrared Integrated Photonics and Remote Sensing (MiPRoS) Laboratory and the TAMU Materials Characterization Facility. The authors appreciate the sponsorships offered by the Texas A&M University (TAMU) Presidential X-Grant and NSF-ERC PATHS-UP Program. This research used resources of the Center for Functional Nanomaterials, which is a U.S. DOE Office of Science User Facility, at Brookhaven National Laboratory.
References
[1] D. Damjanovic, P. Muralt, N. Setter. Ferroelectric sensors. IEEE Sens. J., 1, 191-206(2001).
[2] A. Chanthbouala, A. Crassous, V. Garcia, K. Bouzehouane, S. Fusil, X. Moya, J. Allibe, B. Dlubak, J. Grollier, S. Xavier, C. Deranlot, A. Moshar, R. Proksch, N. D. Mathur, M. Bibes, A. Barthelemy. Solid-state memories based on ferroelectric tunnel junctions. Nat. Nanotechnol., 7, 101-104(2012).
[3] D. Sando, Y. Yang, C. Paillard, B. Dkhil, L. Bellaiche, V. Nagarajan. Epitaxial ferroelectric oxide thin films for optical applications. Appl. Phys. Rev., 5, 041108(2018).
[4] C. Xiong, W. H. P. Pernice, J. H. Ngai, J. W. Reiner, D. Kumah, F. J. Walker, C. H. Ahn, H. X. Tang. Active silicon integrated nanophotonics: ferroelectric BaTiO3 devices. Nano Lett., 14, 1419-1425(2014).
[5] N. Bloembergen. Nonlinear Optics(1996).
[6] A. Hermans, C. Kieninger, K. Koskinen, A. Wickberg, E. Solano, J. Dendooven, M. Kauranen, S. Clemmen, M. Wegener, C. Koos, R. Baets. On the determination of χ(2) in thin films: a comparison of one-beam second-harmonic generation measurement methodologies. Sci. Rep., 7, 44581(2017).
[7] L. E. Myers, R. C. Eckardt, M. M. Fejer, R. L. Byer, W. R. Bosenberg, J. W. Pierce. Quasi-phase-matched optical parametric oscillators in bulk periodically poled LiNbO3. J. Opt. Soc. Am. B, 12, 2102-2116(1995).
[8] M. A. Belkin, F. Capasso, A. Belyanin, D. L. Sivco, A. Y. Cho, D. C. Oakley, C. J. Vineis, G. W. Turner. Terahertz quantum-cascade-laser source based on intracavity difference-frequency generation. Nat. Photonics, 1, 288-292(2007).
[9] H. Jin, F. M. Liu, P. Xu, J. L. Xia, M. L. Zhong, Y. Yuan, J. W. Zhou, Y. X. Gong, W. Wang, S. N. Zhu. On-chip generation and manipulation of entangled photons based on reconfigurable lithium-niobate waveguide circuits. Phys. Rev. Lett., 113, 103601(2014).
[10] S. Arahira, N. Namekata, T. Kishimoto, H. Yaegashi, S. Inoue. Generation of polarization entangled photon pairs at telecommunication wavelength using cascaded χ(2) processes in a periodically poled LiNbO3 ridge waveguide. Opt. Express, 19, 16032-16043(2011).
[11] O. Alibart, V. D’Auria, M. De Micheli, F. Doutre, F. Kaiser, L. Labonté, T. Lunghi, É. Picholle, S. Tanzilli. Quantum photonics at telecom wavelengths based on lithium niobate waveguides. J. Opt., 18, 104001(2016).
[12] J. Wang, J. B. Neaton, H. Zheng, V. Nagarajan, S. B. Ogale, B. Liu, D. Viehland, V. Vaithyanathan, D. G. Schlom, U. V. Waghmare, N. A. Spaldin, K. M. Rabe, M. Wuttig, R. Ramesh. Epitaxial BiFeO3 multiferroic thin film heterostructures. Science, 299, 1719-1722(2003).
[13] K. Suzuki, D. Fu, K. Nishizawa, T. Miki, K. Kato. Ferroelectric property of alkoxy-derived YMnO3 films crystallized in argon. Jpn. J. Appl. Phys., 42, 5692-5695(2003).
[14] S. Raghavan, T. Schumann, H. Kim, J. Y. Zhang, T. A. Cain, S. Stemmer. High-mobility BaSnO3 grown by oxide molecular beam epitaxy. APL Mater., 4, 016106(2016).
[15] R. A. McKee, F. J. Walker, M. F. Chisholm. Physical structure and inversion charge at a semiconductor interface with a crystalline oxide. Science, 293, 468-471(2001).
[16] C. Dubourdieu, J. Bruley, T. M. Arruda, A. Posadas, J. Jordan-Sweet, M. M. Frank, E. Cartier, D. J. Frank, S. V. Kalinin, A. A. Demkov, V. Narayanan. Switching of ferroelectric polarization in epitaxial BaTiO3 films on silicon without a conducting bottom electrode. Nat. Nanotechnol., 8, 748-754(2013).
[17] B. W. Wessels. Ferroelectric epitaxial thin films for integrated optics. Ann. Rev. Mater. Res., 37, 659-679(2007).
[18] D. M. Gill, C. W. Conrad, G. Ford, B. W. Wessels, S. T. Ho. Thin-film channel waveguide electro-optic modulator in epitaxial BaTiO3. Appl. Phys. Lett., 71, 1783-1785(1997).
[19] Y. Garbovskiy, A. Glushchenko. Optical/ferroelectric characterization of BaTiO3 and PbTiO3 colloidal nanoparticles and their applications in hybrid materials technologies. Appl. Opt., 52, E34-E39(2013).
[20] M. K. Trivedi, G. Nayak, S. Patil, R. M. Tallapragada, O. Latiyal, S. Jana. Impact of biofield treatment on atomic and structural characteristics of barium titanate powder. Indus. Eng. Manage., 4, 166(2015).
[21] P. T. Lin, Z. Liu, B. W. Wessels. Ferroelectric thin film photonic crystal waveguide and its electro-optic properties. J. Opt. A, 11, 075005(2009).
[22] A. Petraru, M. Siegert, M. Schmid, J. Schubert, Ch. Buchal. Ferroelectic BaTiO3 thin film optical waveguide modulators. Ferroelectric Thin Films X, 688, 279-284(2002).
[23] T. Zhao, H. Lu, F. Chen, G. Yang, Z. Chen. Stress-induced enhancement of second-order nonlinear optical susceptibilities of barium titanate films. J. Appl. Phys., 87, 7448-7451(2000).
[24] M. B. Lee, M. Kawasaki, M. Yoshimoto, H. Koinuma. Heteroepitaxial growth of BaTiO3 films on Si by pulsed laser deposition. Appl. Phys. Lett., 66, 1331-1333(1995).
[25] M. H. M. Hsu, D. Van Thourhout, M. Pantouvaki, J. Meersschaut, T. Conard, O. Richard, H. Bender, P. Favia, M. Vila, R. Cid. Controlled orientation of molecular-beam-epitaxial BaTiO3 on Si (001) using thickness engineering of BaTiO3 and SrTiO3 buffer layers. Appl. Phys. Express, 10, 065501(2017).
[26] T. Zhao, Z. H. Chen, F. Chen, W. S. Shi, H. B. Lu, G. Z. Yang. Enhancement of second-harmonic generation in BaTiO3/SrTiO3 superlattices. Phys. Rev. B, 60, 1697-1700(1999).
[27] E. Kim, A. Steinbrück, M. T. Buscaglia, V. Buscaglia, T. Pertsch, R. Grange. Second-harmonic generation of single BaTiO3 nanoparticles down to 22 nm diameter. ACS Nano, 7, 5343-5349(2013).
[28] B. Bihari, J. Kumar, G. T. Stauf, P. C. Van Buskirk, C. S. Hwang. Investigation of barium titanate thin films on MgO substrates by second-harmonic generation. J. Appl. Phys., 76, 1169-1174(1994).
[29] F. Capasso, R. Paiella, R. Martini, R. Colombelli, C. Gmachl, T. L. Myers, M. S. Taubman, R. M. Williams, C. G. Bethea, K. Unterrainer, H. Y. Hwang, D. L. Sivco, A. Y. Cho, A. M. Sergent, H. C. Liu, E. A. Whittaker. Quantum cascade lasers: ultrahigh-speed operation, optical wireless communication, narrow linewidth, and far-infrared emission. IEEE J. Quantum Electron., 38, 511-532(2002).
[30] P. T. Lin, H.-Y. G. Lin, Z. Han, T. Jin, R. Millender, L. C. Kimerling, A. Agarwal. Label-free glucose sensing using chip-scale mid-infrared integrated photonics. Adv. Opt. Mater., 4, 1755-1759(2016).
[31] D. Farrah, J. Bernard-Salas, H. W. W. Spoon, B. T. Soifer, L. Armus, B. Brandl, V. Charmandaris, V. Desai, S. Higdon, D. Devost, J. Houck. High-resolution mid-infrared spectroscopy of ultraluminous infrared galaxies. Astrophys. J., 667, 149-169(2007).
[32] P. R. Christensen, B. M. Jakosky, H. H. Kieffer, M. C. Malin, H. Y. McSween, K. Nealson, G. L. Mehall, S. H. Silverman, S. Ferry, M. Caplinger, M. Ravine. The thermal emission imaging system (THEMIS) for the Mars 2001 Odyssey Mission. Space Sci. Rev., 110, 85-130(2004).
[33] T. Jin, J. Zhou, H.-Y. Lin, P. T. Lin. Mid-infrared chalcogenide waveguides for real-time and non-destructive volatile organic compounds detection. Anal. Chem., 91, 817-822(2018).
[34] J. Zhou, P. T. Lin. Mid-infrared multi-spectral detection for real-time and non-invasive analysis of structure and composition of materials. ACS Sens., 3, 1322-1328(2018).
[35] H. D. Megaw. Origin of ferroelectricity in barium titanate and other perovskite-type crystals. Acta Crystallogr., 5, 739-749(1952).
[36] S. A. Denev, T. T. A. Lummen, E. Barnes, A. Kumar, V. Gopalan. Probing ferroelectrics using optical second harmonic generation. J. Am. Ceram. Soc., 94, 2699-2727(2011).
[37] O. Diéguez, K. M. Rabe, D. Vanderbilt. First-principles study of epitaxial strain in perovskites. Phys. Rev. B, 72, 144101(2005).