Abstract
Lens-less Fourier-transform holography has been actively studied because of its simple optical structure and its single-shot recording. However, a low-contrast interferogram between the reference and object waves limits its signal to noise ratio. Here, multi-reference lens-less Fourier-transform holography with a Greek-ladder sieve array is proposed in the experiment and demonstrated effectively to improve the signal to noise ratio. The key technique in our proposed method is a Greek-ladder sieve array, which acts as not only a wave-front modulator but also a beam splitter. With advantages of the common path, single shot, and no need for a lens, this system has enormous potential in imaging and especially in extreme ultraviolet and soft X-ray holography.Holography is a promising three-dimensional imaging technique with a wide range of applications[1–3]. Since it was invented by Gabor[4], researchers have never stopped pursuing simple configurations, few operation steps, as well as high resolution. Although Gabor holographic recording is common-path, it cannot get rid of the direct and the conjugate terms from the real image[5]. Then, off-axis holography is invented to separate the undesired terms, but its recording is realized by two beam paths[6]. In-line phase-shifting holography is also proposed by two beam paths and needs more than one exposure to remove these distractors[7]. Fourier-transform holography (FTH) can avoid the above drawbacks in that its recording not only is a common path, but also takes full advantage of the charge coupled device (CCD) target[8]. With the tremendous development of free-electron lasers and relative diagnostic technologies[9], no need for lens, single light path, and single exposure in FTH are great advantages in applications in high-resolution extreme ultraviolet (EUV) and X-ray holography[10]. In fact, FTH has been used to image nanoscale structures, such as magnetic samples[11,12] and biological objects[13,14], as well as ultra-fast dynamics[15,16].
The optical structure of lens-less FTH usually consists of the following parts: a coherent light source, a plane wave incident on the object, a reference pinhole channeled around the object, and a CCD to record the interference pattern. Essentially, the pinhole plays as a point source emitting a reference spherical wave with the same curvature on the CCD screen, which is the key point in FTH[8]. In addition, the resolution is proportional to the size of the reference pinhole, which is limited by the current machining precision. Taking into account a plane wave incidence, the pinhole is small enough that it produces a low-intensity reference beam, which will result in a low-contrast interference pattern. In order to improve the signal to noise ratio (SNR), the intensity of the reference beam needs to be enhanced. Apart from adding a regular lens to focus, another effective method is to introduce multi-reference pinholes[17], as theoretically demonstrated in constructive multi-interference of emitted waves through the lens-free technique, which can improve the system SNR (coupling-to-loss)[18–20]. In that way, the SNR of the reconstructed image is proportional to the square root of the number of pinholes[21].
Based on this concept, in order to enhance the contrast of interference pattern and simultaneously improve the energy utilization, here, a Greek-ladder sieve array is firstly introduced into FTH and emerges as multiple high-intensity spherical reference waves. In the experiment, the photon-sieve (PS) array is placed between the light source and object plane, and multi-reference pinholes coincide with these focal spots of the Greek-ladder sieve array, which are amplitude-only diffractive lenses[22,23] and have the ability to modulate the wave-front, working as phase shifter[24,25] or structured-light generator[26,27]. In traditional FTH, the reference spherical beam can be generated by a condenser lens or some pinholes[21,28]. The former cannot be directly used for soft X-ray due to its strong absorption; the latter leads to the low-intensity reference wave and further results in the low-contrast SNR. The proposed method takes advantage of an amplitude-only Greek-ladder sieve to focus the incident wave and then emerges as a reference sphere wave. More importantly, a Greek-ladder sieve can generate multiple axial foci with different lateral resolutions, which is more suitable for a reference pinhole of a different size. After all, the system resolution is of the same order as the size of the reference pinhole. In this Letter, the Greek-ladder sieve array splits the incident plane wave into four different parts: one beam in the center is referred to as the object wave, and other three are referred to as the multi-reference waves. Multiple high-intensity reference waves meet the requirements of the high-contrast interference pattern, which is very beneficial for reconstruction and easy for obtaining a high-quality image.
Sign up for Chinese Optics Letters TOC. Get the latest issue of Chinese Optics Letters delivered right to you!Sign up now
To demonstrate the resolution of the FTH and further enhance it, a key element in our system is the Greek-ladder sieve array. As depicted in Fig. 1, the PS array mainly consists of three amplitude-only Greek-ladder sieves fabricated by photolithography, and the quasi-triangle transparent area in the middle is referred to as the illumination source. The three identical Greek-ladder sieves have the same diameter of 5.2 mm and bifocal lengths of and . The value , which approximately equals the square root of two[23,24]. Figures 1(c) and 1(d) show that the bifocal spots, respectively, have a diameter of 15.2 μm and 21.5 μm, and their ratio is also equal to the square root of two.
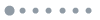
Figure 1.(a) Schematic of a Greek-ladder sieve array, in which the white area is transparent; (b) the intensity distribution of one Greek-ladder sieve along the axis; (c) the first Airy spot with a diameter of 15.2 μm; (d) the second Airy spot with a diameter of 21.5 μm.
In Fig. 2, a He–Ne laser with a wavelength of 632.8 nm is used as the light source. The laser beam is collimated and expanded by a collimator with a magnification factor of 10. In the experiment, the three Airy spots with the same diameter of 15.2 μm at the first focal plane simultaneously pass through three reference pinholes and then are referred to as the three reference spherical waves. In the object plane, there are three 25 μm diameter pinholes uniformly distributed in a circle with a radius of 3 mm, filtering these focal spots of the Greek-ladder sieve array and emerging as spherical reference waves. The transparent test object, as shown in detail in Fig. 3(a), consists of four lines ranging in width from 50 μm to 300 μm, distributed in a square with a side of 0.6 mm. The diffracted field of the test object interferes with the three independent reference waves. Then, the interference pattern is recorded by a CCD ( pixels with size of ), which is placed at a distance of from the object plane. The recording distance is 110 mm in our experiment. The test object can be easily reconstructed by direct Fourier transformation of the hologram.
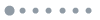
Figure 2.Schematic of multiple reference lens-less Fourier-transform holography, in which the Greek-ladder sieve array plays the role of splitter, and condenser lenses and the three pinholes work as filters and quasi-point light sources on the object plane.
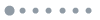
Figure 3.(a) Test object with four transparent lines, anti-clockwise from the upper-right corner: , , , and ; (b) hologram on the CCD with ; (c) reconstructed image (); (d) enlargement of one subgraph (); (e) weighted-average reconstruction from the three subgraphs in (c) ().
Figure 3(b) shows the hologram with pixels extracted from the original data on the CCD screen. The reconstructed images are indicated in Fig. 3(c), in which they uniformly rotate around the center of the tri-reference pinholes. Figure 3(d) depicts the enlargement of the subgraph in the red box in Fig. 3(c). In this image, the smallest line can be resolved as expected. Weighted-average reconstruction is shown in Fig. 3(e), which is the average of the subfigures in the three red boxes from Fig. 3(c). Compared with Fig. 3(d), tri-reference FTH improves the image contrast and forms a sharper edge of the lines than the traditional FTH with only one reference point. In Fig. 3(e), all the test lines can be solved clearly. Because the smallest line width is , we can say the system resolution is better than 50 μm.
In order to guarantee that three reference pinholes coincide with these focal spots, the expanded beam must be an ideal wave-front, which can be verified by a lateral shearing interferometer. Apart from a little difficulty in the optical path adjustment, the whole experimental process is simple, smooth, and fast. Compared with traditional FTH, the proposed method greatly enhances the interference contrast by use of multi-reference pinholes and high-intensity reference beams. Another benefit is that the Greek-ladder sieve provides multi-focal spots of different sizes, which can be fully used to adjust the interference contrast with respect to a fixed-size reference pinhole. After all, a high SNR hologram is more helpful in reconstructing the test object.
In conclusion, a multi-reference FTH with a Greek-ladder sieve array has been demonstrated and carried out in the experiment. This system contains a coherent light source, a PS array, and a detector. The Greek-ladder sieve array splits the light source into four wave-fronts, three of which are convergent spherical waves and filtered by three reference pinholes at the object plane; the last beam in the center is referred to as the illumination source. Once the interference pattern is recorded by the detector, the test object can be reconstructed by direct Fourier transformation. Multi-reference FTH not only greatly improves the SNR of the reconstructed image, but also enhances the anti-jamming ability of the optical system. The experimental results verify the effectiveness of the proposed method. Due to the amplitude-only diffractive lens and single-shot recording path, it will have great potential in EUV and soft X-ray holography.
References
[1] C. Knox. Science, 153, 989(1966).
[2] J. C. Solem, G. C. Baldwin. Science, 218, 229(1982).
[3] C. J. Mann, L. Yu, C. M. Lo, M. K. Kim. Opt. Express, 13, 8693(2005).
[4] D. Gabor. Nature, 161, 777(1948).
[5] D. Gabor. Proc. Roy. Soc. A, 197, 454(1949).
[6] E. N. Leith, J. Upatniek. J. Opt. Soc. Am, 52, 1123(1962).
[7] I. Yamaguchi, T. Zhang. Opt. Lett., 22, 1268(1997).
[8] G. W. Stroke. Appl. Phys. Lett., 6, 201(1965).
[9] F. Brandi, L. A. Gizzi. High Power Laser Sci. Eng., 7, e26(2019).
[10] I. McNulty, J. Kirz, C. Jacobsen, E. H. Anderson, M. R. Howells, D. P. Kern. Science, 256, 1009(1992).
[11] S. Eisebitt, J. Lüning, W. F. Schlotter, M. Lörgen, O. Hellwig, W. Eberhardt, J. Stöhr. Nature, 432, 885(2004).
[12] D. L. Zhu, M. Guizar-Sicairos, B. Wu, A. Scherz, Y. Acremann, T. Tyliszczak, P. Fischer, N. Friedenberger, K. Ollefs, M. Farle, J. R. Fienup. Phys. Rev. Lett., 105, 043901(2010).
[13] E. Guehrs, C. M. Gunther, R. Konnecke, B. Pfau, S. Eisebitt. Opt. Express, 17, 6710(2009).
[14] A. P. Mancuso, T. Gorniak, F. Staier, O. M. Yefanov, R. Barth, C. Christophis, B. Reime, J. Gulden, A. Singer, M. E. Pettit, T. Nisius. New J. Phys., 12, 35003(2010).
[15] G. O. Williams, A. I. Gonzalez, S. Künzel, L. Li, M. Lozano, E. Oliva, B. Iwan, S. Daboussi, W. Boutu, H. Merdji, M. Fajardo. Opt. Lett., 40, 3205(2015).
[16] D. Gauthier, M. Guizar-Sicairos, X. Ge, W. Boutu, B. Carré, J. R. Fienup, H. Merdji. Phys. Rev. Lett., 105, 093901(2010).
[17] J. D. Gaskill, J. W. Goodman. Proc. IEEE, 57, 823(1969).
[18] Y. Zhou, Z. Chen, J. T. Shen. Phys. Rev. A, 95, 043832(2017).
[19] Z. Chen, Y. Zhou, J. T. Shen. Opt. Lett., 42, 887(2017).
[20] Y. Shen, Z. Chen, Y. He, Z. Li, J. T. Shen. J. Opt. Soc. Am. B, 35, 607(2018).
[21] W. F. Schlotter, R. Rick, K. Chen, A. Scherz, J. Stöhr, J. Lüning, S. Eisebitt, C. Günther, W. Eberhardt, O. Hellwig, I. McNulty. Appl. Phys. Lett., 89, 163112(2006).
[22] J. Y. Zhang. Opt. Express, 23, 30308(2015).
[23] S. Y. Xu, J. Zhang, S. Zhou, Y. Ma, S. Wang, Y. Zhang, J. Xie, J. Zhu. Appl. Opt., 57, 1993(2018).
[24] J. Xie, J. Y. Zhang, Y. L. Zhang, S. L. Zhou, J. Q. Zhu. Appl. Phys. Lett., 112, 151906(2018).
[25] Y. Y. Bian, Y. J. Liu, L. Jiang. Chin. Opt. Lett., 16, 093301(2018).
[26] X. P. Zhang, J. Y. Zhang, S. L. Zhou, D. Liu, J. Q. Zhu. Laser Phys. Lett., 16, 025002(2019).
[27] H. Zang, C. Zheng, Q. Fan, C. Wang, L. Wei, L. Cao, X. Wang, E. Liang. Chin. Opt. Lett., 16, 080501(2018).
[28] M. Gustafsson, M. Sebesta, B. Bengtsson, S. G. Pettersson, P. Egelberg, T. Lenart. Opt. Laser Eng., 41, 553(2004).