
- Chinese Optics Letters
- Vol. 21, Issue 2, 023602 (2023)
Abstract
1. Introduction
Metasurfaces[1], which are made of micro-optical antennas with versatile shapes and arrangements, circumvent the fabrication problems with one dimension reduced to the subwavelength scale, without sacrificing powerful functionalities. The research fields of metasurfaces can be roughly divided into two categories: classical and quantum domains. In the classical domain, the invisible cloak carpet[2], metalens[3,4], holography[5], nonlinear optics[6], and so on have been demonstrated. On the other hand, in the quantum domain, researchers have developed highly integrated quantum optical elements, ranging from quantum sources based on metalens[7], quantum manipulation[8–10] and applications[11–14], quantum vacuum engineering of quantum emitters[15–17], and so on. Interested readers can refer to several comprehensive reviews published recently[18,19].
Among all, researchers care about the abundant capabilities embedded in only one metasurface sample. Degrees of freedom of light, such as wavelength[20–22], incident[23–25] or output directions[26], orbital angular momentum[27–29], and polarizations[30–32], have been explored for realizing multiple functionalities. The state of light passing through metasurfaces is dependent on the degrees of freedom of incident photons. Combining the propagation phase and geometric phase of metasurfaces, independent and arbitrary phase can be encoded into orthogonal polarization bases[32,33]. Furthermore, relying on the subwavelength-scale pixels of metasurfaces and the interference principle, the complete decoupling of near- and far-field patterns for orthogonal polarization bases is possible[30]. Meanwhile, independent far-field patterns encoded into two wavelengths have also been confirmed feasible[34]. However, near- and far-field patterns decoupled completely for two wavelengths simultaneously have yet to be demonstrated.
In this paper, we utilize the different dispersion properties of propagation phase and geometric phase to achieve
Sign up for Chinese Optics Letters TOC. Get the latest issue of Chinese Optics Letters delivered right to you!Sign up now
2. Principles
The phase modulation mechanisms of metasurfaces mainly consist of two types: propagation phase and geometric phase. The propagation phase is related to wavelength and has nothing to do with the rotation angles of meta-atoms. The opposite is true for the geometric phase. Based on this, we can find six types of pillars whose propagation phase difference at 1064 nm and 1550 nm covers the full range of
Figure 1.Schematic of dual-wavelength near- and far-field decoupling metasurfaces.
Figure 2.(a) Structure parameters of a meta-atom. (b) Full-wave simulations varying with width and length. The colormap represents the phase given by a meta-atom. (c) Phase varying with theta for six meta-atoms at the wavelength of 1064 nm. (d) Phase varying with theta for the same six meta-atoms at the wavelength of 1550 nm. (e) Phase difference between 1064 nm and 1550 nm for six meta-atoms. (f) Cross-polarization conversion efficiency for six meta-atoms at the wavelength of 1064 nm. (g) Cross-polarization conversion efficiency for the same six meta-atoms at the wavelength of 1550 nm.
Although the amplitude and phase of incident light can be separately controlled by metasurfaces easily, it is difficult to simultaneously control the amplitude and phase of incident light by a single meta-atom. According to the principle of interference, the phase and amplitude of incident light in a super-pixel can be manipulated at the same time as required when only the phases of constituent meta-atoms are demanded. As shown in Fig. 3(a), two groups of meta-atoms are placed across each other. For a single meta-atom, the output electric field in the scalar form is
Figure 3.(a) Meta-atom arrangement of 2 × 2 at a super-pixel. The 1 and 2 possess different structural parameters. (b) The design process of dual-wavelength near- and far-field decoupling metasurfaces. The ① represents the GS algorithm, ② represents one-to-four algorithm, and ③ represents getting structural parameters from dual-wavelength phase distribution.
Then, we can conclude that to achieve utmost visibility the cross-polarization conversion efficiency is not necessarily equal to unity but needed to be the same. This relaxes the constrains for the pillars.
The design process is shown in Fig. 3(b). Firstly, the patterns of two wavelengths in the near field and far field are determined, respectively, and the far-field patterns are inverted by the Gerchberg–Saxton (GS) algorithm to get the near-field phase, as shown by ① in Fig. 3(b). After obtaining the near-field phase and intensity of the super-pixel, the one-to-four algorithm is designed based on the formula above: the intensity and phase of one super-pixel can be achieved by four pixels. Finally, after the phase distributions of wavelengths of 1064 nm and 1550 nm are obtained, the corresponding phase requirements are realized by using the found meta-atoms, which determine the length, width, and rotation angle distribution of the metasurface.
3. Simulation Results
3.1. Near-field pattern and far-field pattern
The size of the metasurface is
Figure 4.Simulation results. (a), (c) Near-field intensity distribution at the distance of 100 nm after the metasurface for 1064 nm and 1550 nm, respectively. (b), (d) Far-field intensity distribution after the metasurface for 1064 nm and 1550 nm, respectively.
3.2. Near-field pattern and focusing
Based on the same principle, we can also design dual-wavelength near-field patterns and arbitrary independent focal lengths. Figures 5(a) and 5(d) show the intensity distribution of the near-field electric field at a distance of 100 nm away from the metasurface, which the light with the incident wavelength of 1064 nm or 1550 nm passes through, respectively. Figures 5(b) and 5(c), respectively, show the focusing effect along with the
Figure 5.Simulation results. (a), (d) Near-field intensity distribution at the distance of 100 nm after the metasurface for 1064 nm and 1550 nm, respectively. (b), (c) Verification of the converging metalens at an incident wavelength of 1064 nm for horizontal and vertical directions, respectively. (e), (f) Verification of the converging metalens at an incident wavelength of 1550 nm for horizontal and vertical directions, respectively.
The above equations suggest that when the NA of the lens is very small, the final output focal length is four times as large as the initial input focal length.
Metasurfaces are wavelength-dependent structures. Using this method, it is easy to design dual-wavelength planar devices with the same or different focal lengths, and there is no need to find wavelength-selective structures in segmented implementation. As shown in Fig. 6(a), each data point represents the situation in which one wavelength is fixed at one initial input focal length, and the initial input focal length of the other wavelength changes from 10 µm to 300 µm. When the initial input focal length of 1064 nm is 10–150 µm, the change of the initial focal length of 1550 nm has a great influence on the final output focal length of 1064 nm, and the relationship between the final output focal length and the initial input focal length for 1064 nm is not four-fold linear. Otherwise, when the initial input focal length of 1064 nm is 150–300 µm, the initial input focal length of 1550 nm has less influence on the final output focal length of 1064 nm, and the final output focal length of 1064 nm is closer to a four-fold relationship with the initial input focal length of 1550 nm. The case is the same when the 1550 nm initial input focal length is unchanged, while the 1064 nm initial input focal length varies. In addition to focusing, the far-field function can also be beam deflection, and the final output deflection angle should be 1/2 as large as the initial design deflection angle. In order to better characterize the focusing performance, Fig. 6(b) shows the focusing efficiency for two wavelengths. The longer the focal length, the higher the focusing efficiency. Figures 6(c) and 6(d) show the sizes of the focusing spot in the
Figure 6.Simulation results. (a) The relationships between final output focus length and initial input focus length. (b) The average efficiency of focusing obtained for 1064 nm and 1550 nm. (c), (d) The FWHMs obtained for horizontal and vertical polarizations for 1064 nm and 1550 nm, respectively. The error bars represent the minimum and maximum values.
4. Discussion
In summary, we show that we can use the different dispersion properties of propagation phase and geometric phase of metasurfaces to achieve complete decoupling of dual-wavelength regulation in terms of phase and intensity. Firstly, we find the pillars whose two-wavelength propagation phase difference covers
The theoretical design provided in this paper can be fully realized experimentally. The highest aspect ratio of the adopted meta-atoms is roughly 8:1, which lies within a reasonable range. Via plasma-enhanced chemical vapour deposition (PECVD), electron beam lithography (EBL), inductively coupled plasma and reactive ion etching (ICP-RIE) etcher, and so on, a complete set of micro/nano fabrication processes makes sample fabrication possible. To the best of our knowledge, this is the first time that simultaneous complete near- and far-field decoupling for two wavelengths is demonstrated. This functionality can not only improve the information density and security of nanostructured metasurfaces, but also stands a good chance of broadening application scenarios such as AR applications and stimulated emission depletion microscopy, in which multi-wavelength capability is necessary. This work is instructive for achieving more sophisticated multi-wavelength and multi-functional meta-devices and realizing useful applications in practical systems.
References
[1] N. Yu, P. Genevet, A. Kats Mikhail, F. Aieta, J.-P. Tetienne, F. Capasso, Z. Gaburro. Light propagation with phase discontinuities: generalized laws of reflection and refraction. Science, 334, 333(2011).
[2] X. Ni, J. Wong Zi, M. Mrejen, Y. Wang, X. Zhang. An ultrathin invisibility skin cloak for visible light. Science, 349, 1310(2015).
[3] S. Wang, P. C. Wu, V.-C. Su, Y.-C. Lai, C. H. Chu, J.-W. Chen, S.-H. Lu, J. Chen, B. Xu, C.-H. Kuan. Broadband achromatic optical metasurface devices. Nat. Commun., 8, 187(2017).
[4] S. Wang, P. C. Wu, V.-C. Su, Y.-C. Lai, M.-K. Chen, H. Y. Kuo, B. H. Chen, Y. H. Chen, T.-T. Huang, J.-H. Wang. A broadband achromatic metalens in the visible. Nat. Nanotechnol., 13, 227(2018).
[5] G. Zheng, H. Mühlenbernd, M. Kenney, G. Li, T. Zentgraf, S. Zhang. Metasurface holograms reaching 80% efficiency. Nat. Nanotechnol., 10, 308(2015).
[6] W. Klein Matthias, C. Enkrich, M. Wegener, S. Linden. Second-harmonic generation from magnetic metamaterials. Science, 313, 502(2006).
[7] L. Li, Z. Liu, X. Ren, S. Wang, V. C. Su, M. K. Chen, C. H. Chu, H. Y. Kuo, B. Liu, W. Zang, G. Guo, L. Zhang, Z. Wang, S. Zhu, D. P. Tsai. Metalens-array-based high-dimensional and multiphoton quantum source. Science, 368, 1487(2020).
[8] T. Stav, A. Faerman, E. Maguid, D. Oren, V. Kleiner, E. Hasman, M. Segev. Quantum entanglement of the spin and orbital angular momentum of photons using metamaterials. Science, 361, 1101(2018).
[9] K. Wang, J. G. Titchener, S. S. Kruk, L. Xu, H. P. Chung, M. Parry, I. I. Kravchenko, Y. H. Chen, A. S. Solntsev, Y. S. Kivshar, D. N. Neshev, A. A. Sukhorukov. Quantum metasurface for multiphoton interference and state reconstruction. Science, 361, 1104(2018).
[10] Q. Li, W. Bao, Z. Nie, Y. Xia, Y. Xue, Y. Wang, S. Yang, X. Zhang. A non-unitary metasurface enables continuous control of quantum photon–photon interactions from bosonic to fermionic. Nat. Photonics, 15, 267(2021).
[11] T. Roger, S. Vezzoli, E. Bolduc, J. Valente, J. J. F. Heitz, J. Jeffers, C. Soci, J. Leach, C. Couteau, N. I. Zheludev, D. Faccio. Coherent perfect absorption in deeply subwavelength films in the single-photon regime. Nat. Commun., 6, 7031(2015).
[12] L. M. Procopio, L. A. Rozema, Z. J. Wong, D. R. Hamel, K. O’Brien, X. Zhang, B. Dakic, P. Walther. Single-photon test of hyper-complex quantum theories using a metamaterial. Nat. Commun., 8, 15044(2017).
[13] J. Zhou, S. Liu, H. Qian, Y. Li, H. Luo, S. Wen, Z. Zhou, G. Guo, B. Shi, Z. Liu. Metasurface enabled quantum edge detection. Sci. Adv., 6, eabc4385(2020).
[14] H. Defienne, B. Ndagano, A. Lyons, D. Faccio. Polarization entanglement-enabled quantum holography. Nat. Phys., 17, 591(2021).
[15] P. K. Jha, X. Ni, C. Wu, Y. Wang, X. Zhang. Metasurface-enabled remote quantum interference. Phys. Rev. Lett., 115, 025501(2015).
[16] P. K. Jha, N. Shitrit, J. Kim, X. Ren, Y. Wang, X. Zhang. Metasurface-mediated quantum entanglement. ACS Photonics, 5, 971(2017).
[17] P. K. Jha, N. Shitrit, X. Ren, Y. Wang, X. Zhang. Spontaneous exciton valley coherence in transition metal dichalcogenide monolayers interfaced with an anisotropic metasurface. Phys. Rev. Lett., 121, 116102(2018).
[18] A. S. Solntsev, G. S. Agarwal, Y. S. Kivshar. Metasurfaces for quantum photonics. Nat. Photonics, 15, 327(2021).
[19] J. Liu, M. Shi, Z. Chen, S. Wang, Z. Wang, S. Zhu. Quantum photonics based on metasurfaces. Opto-Electron. Adv., 4, 09200092(2021).
[20] O. Eisenbach, O. Avayu, R. Ditcovski, T. Ellenbogen. Metasurfaces based dual wavelength diffractive lenses. Opt. Express, 23, 3928(2015).
[21] J. Ding, N. Xu, H. Ren, Y. Lin, W. Zhang, H. Zhang. Dual-wavelength terahertz metasurfaces with independent phase and amplitude control at each wavelength. Sci. Rep., 6, 34020(2016).
[22] J. Ding, S. An, B. Zheng, H. Zhang. Multiwavelength metasurfaces based on single-layer dual-wavelength meta-atoms: toward complete phase and amplitude modulations at two wavelengths. Adv. Opt. Mater., 5, 1700079(2017).
[23] Z. Shi, A. Y. Zhu, Z. Li, Y.-W. Huang, W. T. Chen, C.-W. Qiu, F. Capasso. Continuous angle-tunable birefringence with freeform metasurfaces for arbitrary polarization conversion. Sci. Adv., 6, eaba3367(2020).
[24] A. Leitis, A. Tittl, M. Liu, B. H. Lee, M. B. Gu, Y. S. Kivshar, H. Altug. Angle-multiplexed all-dielectric metasurfaces for broadband molecular fingerprint retrieval. Sci. Adv., 5, eaaw2871(2019).
[25] S. M. Kamali, E. Arbabi, A. Arbabi, Y. Horie, M. Faraji-Dana, A. Faraon. Angle-multiplexed metasurfaces: encoding independent wavefronts in a single metasurface under different illumination angles. Phys. Rev. X, 7, 041056(2017).
[26] Y.-J. Gao, X. Xiong, Z. Wang, F. Chen, R.-W. Peng, M. Wang. Simultaneous generation of arbitrary assembly of polarization states with geometrical-scaling-induced phase modulation. Phys. Rev. X, 10, 031035(2020).
[27] Y. Guo, S. Zhang, M. Pu, Q. He, J. Jin, M. Xu, Y. Zhang, P. Gao, X. Luo. Spin-decoupled metasurface for simultaneous detection of spin and orbital angular momenta via momentum transformation. Light Sci. Appl., 10, 63(2021).
[28] H. Ren, X. Fang, J. Jang, J. Bürger, J. Rho, S. A. Maier. Complex-amplitude metasurface-based orbital angular momentum holography in momentum space. Nat. Nanotechnol., 15, 948(2020).
[29] H. Ren, G. Briere, X. Fang, P. Ni, R. Sawant, S. Héron, S. Chenot, S. Vézian, B. Damilano, V. Brändli. Metasurface orbital angular momentum holography. Nat. Commun., 10, 2986(2019).
[30] M. Liu, W. Zhu, P. Huo, L. Feng, M. Song, C. Zhang, L. Chen, H. J. Lezec, Y. Lu, A. Agrawal. Multifunctional metasurfaces enabled by simultaneous and independent control of phase and amplitude for orthogonal polarization states. Light Sci. Appl., 10, 107(2021).
[31] Q. Fan, M. Liu, C. Zhang, W. Zhu, Y. Wang, P. Lin, F. Yan, L. Chen, H. J. Lezec, Y. Lu. Independent amplitude control of arbitrary orthogonal states of polarization via dielectric metasurfaces. Phys. Rev. Lett., 125, 267402(2020).
[32] J. B. Mueller, N. A. Rubin, R. C. Devlin, B. Groever, F. Capasso. Metasurface polarization optics: independent phase control of arbitrary orthogonal states of polarization. Phys. Rev. Lett., 118, 113901(2017).
[33] H. Wang, Z. Zhang, K. Zhao, W. Liu, P. Wang, Y. Lu. Independent phase manipulation of co- and cross-polarizations with all-dielectric metasurface. Chin. Opt. Lett., 19, 053601(2021).
[34] G. Yoon, J. Kim, J. Mun, D. Lee, K. T. Nam, J. Rho. Wavelength-decoupled geometric metasurfaces by arbitrary dispersion control. Commun. Phys., 2, 129(2019).
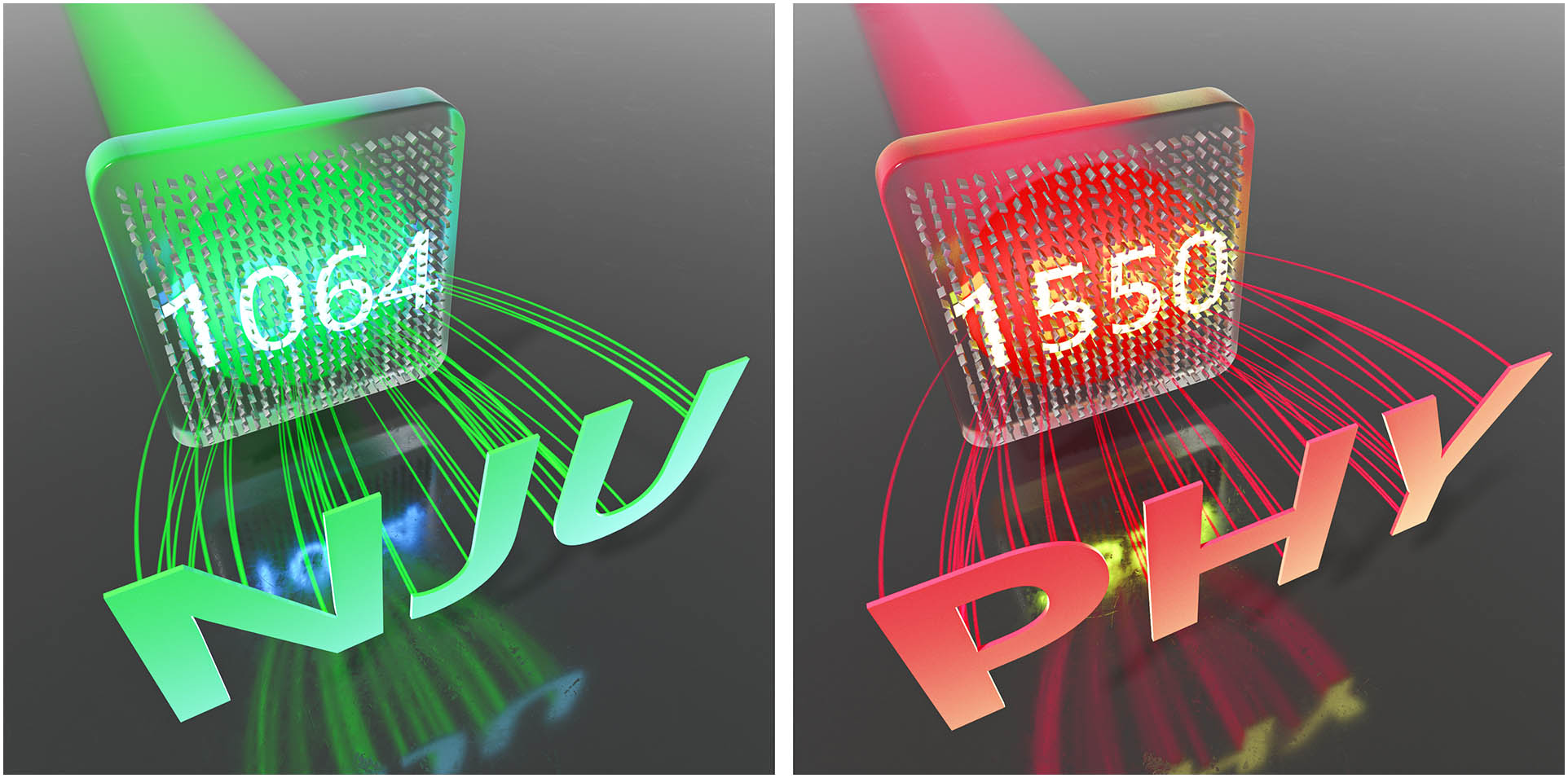
Set citation alerts for the article
Please enter your email address