Abstract
In this Letter, we experimentally demonstrate a full-duplex transmission system of IEEE 802.11ac-compliant multiple-input multiple-output (MIMO) signals over a 2-km 7-core fiber for in-building wireless local-area network (WLAN) distributed antenna systems. For full-duplex MIMO demonstration, the crosstalk impacts of both fiber-transmission-only and optic-wireless transmission situation are evaluated. The results indicate that the impact of crosstalk on radio-over-fiber (ROF) link performance is not significant and the quality of the cascaded multi-core fiber and wireless channel is mainly determined by the wireless part. To further improve the system capacity, polarization multiplexing (PolMux) technology is employed to achieve a full-duplex MIMO over a single 7-core fiber. Although employing the PolMux method will slightly decrease the EVM and condition number performance as opposed to a non-PolMux MCF system, it is still a competitive solution in large optical connection demand scenarios that require a low cost.Distributed antenna systems (DASs) using radio-over-fiber (RoF) techniques have been recognized as a promising solution for in-building and hotspot wireless coverage[1–3]. With a shared RoF-DAS infrastructure, it is preferable to simultaneously distribute wireless local-area network (WLAN) signals as an important complement to cellular signals. Recently, several commercial WLAN products compliant with IEEE 802.11ac standards have become available. In the physical layer, 802.11ac further enhances the RF channel bandwidth up to 160 MHz, the modulation level up to 256-ary quadrature amplitude modulation (256-QAM), and the spatial-stream number up to 8 for multi-user multiple-input multiple-output (MIMO)[4]. With these features, 802.11ac can achieve at least 1 Gb/s rates for the multiple user case and at least 500 Mb/s rates for the single user case, which represents an increase of five times the maximum achievable rates compared to 802.11n. To distribute scalable 802.11ac signals using an RoF DAS architecture, up to 8 weakly correlated channels are required for MIMO transmission. Obviously, several parallel single-mode fiber (SMF) links are not an efficient solution for scalable RF signal distribution use.
Space-division multiplexing (SDM) over a multi-core fiber (MCF) has been under intensive research to increase the capacity per cross-sectional area of the fiber[5,6]. MCF has been acknowledged because of its high space efficiency, especially in large optical connection demand scenarios. More recently, the suitability of SDM in MCF was evaluated for long term evolution (LTE)-advanced optical fronthaul systems in 4-core MCF[7,8]. The work demonstrated a full-duplex LTE-MIMO system and discussed the crosstalk penalty caused by the bending radius and the impact of same or counter-propagations in fiber-transmission-only environments. As the RoF DAS architecture is significant in providing wireless coverage, the optic-wireless link joint impact is an important issue to be evaluated for wireless use. On the other hand, the polarization multiplexing (PolMux) method can effectively double the spectral efficiency and could carry more channels on a signal fiber. Researchers have employed PolMux methods for MIMO-fiber transmission systems[9,10]. In this Letter, the optic-wireless link impact of the crosstalk and the performance of using PolMux in MCF to further improve the capacity will be herein evaluated.
Due to power and cost considerations in real implementations, most of commercially available 802.11ac products support 3 or 6 spatial streams. Hence, two scenarios are considered in this Letter: full-duplex 802.11ac-compliant 3-spatial stream with wireless transmission (Scenario1), and 6-spatial stream with PolMux transmission (Scenario2) over a 2 km 7-core MCF. Note that the medium-access control protocol of the IEEE 802.11 standards defines the maximum time delay in the physical layer, thus commonly limiting fiber reaches lower than 2 km. The inner core is reserved as a dedicated control channel for the remote antenna unit (RAU)[11]. The impacts of the crosstalk on the link dynamic range and optical power sensitivity were also evaluated.
Sign up for Chinese Optics Letters TOC. Get the latest issue of Chinese Optics Letters delivered right to you!Sign up now
Scenario 1: Full-duplexMIMO transmission scenario
Figure 1 shows the experimental setup of full-duplex IEEE 802.11ac-compliant MIMO signals over a 2-km 7-core fiber and 1-m wireless. The homogeneous 7-core MCF used in our experiments has one inner core and six outer cores in a hexagonal layout[12]. With the help of fan-in/fan-out fiber devices, the 7-core MCF can support up to 7 parallel weakly coupled channels. The dispersions of the 7 cores are measured at 1550 nm as 17.12, 17.03, 17.05, 17.13, 16.78, 17.61, and 16.88 (ps/nm/km), respectively, which is a similar level to the SMF. In this Letter, 2 km MCF was used and the dispersion effect is negligible. In order to reduce the crosstalk between uplink and downlink, Cores 1, 5, and 6 were assigned to MIMO channels in the downlink, and Cores 2, 3, and 7 were assigned for another MIMO channels in the uplink. The parallel analog optical links were enabled by six low-cost 6-GHz analog directly modulated optical transceivers at a wavelength of around 1550 nm. The optical losses of each core are listed in Fig. 1, and the lasers’ output power is . We tried to match low output power lasers to low optical loss cores to further balance out the six links, which means that the lowest received optical power before the photodiode was around 1 dBm. Therefore, the received optical powers before the photodiodes are around 1–2 dBm, which is in the best working range, according to the measurement results. Two kinds of setups are discussed in Scenario 1. In Setup 1, RF cables were used to connect the PDs and the analyzer modules, aiming at evaluating the performance of the multicore fiber only. In Setup 2, we further considered the fiber-wireless hybrid transmission scenario, using omnidirectional antennas (5-dBi gain) to build a 1 m wireless environment.
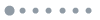
Figure 1.Experimental setup for full-duplex IEEE 802.11ac-compliant MIMO over 2-km 7-core fiber and 1-m wireless. Insets: photos of experimental environment and electrical spectra of WLAN signals after MCF transmission.
Keysight M9381 PXIe vector signal generators (VSGs) and M9391 PXIe vector signal analyzers (VSAs) were used to build the MIMO WLAN physical layer testing platform. IEEE 802.11ac-compliant signals in the format of the seventh modulation and coding scheme (MCS7) (i.e. 64QAM-OFDM, 5/6 coding rate) and MSC8 (256QAM-OFDM, 3/4 coding rate) with 80 MHz bandwidth were generated by Keysight WLAN7617B software and analyzed by Keysight 89601B vector signal analysis software.
Setup 1: Fiber transmission only
For the fiber-transmission-only setup, three cases were considered: (1) nonadjacent Cores 1, 5, and 6 used to transmit one group of MIMO signals; (2) Cores 1, 5, and 6 and Cores 2, 3, and 7 MIMO signals used to transmit two groups of MIMO signals in two opposite propagation directions; (3) Cores 1, 5, and 6 and Cores 2, 3, and 7 used to transmit two groups of MIMO signals in the same propagation direction.
To evaluate the crosstalk impact over the entire 6 GHz frequency range, the condition number of the MIMO channel matrix as a function of the RF carrier frequency was measured, as shown in Fig. 2. The condition number is an important indicator of the MIMO channel state. In general, a condition number of smaller than 20 dB (i.e., 10) is sufficient to support accepted wireless MIMO transmissions in a typical in-building wireless environment[13]. In order to attain the crosstalk between the two groups of MIMO signals, we applied the MIMO testing mode in Case 3. Within the range from 0–6 GHz, the condition number fluctuations are relatively small in all three cases. The results clearly show that Case 3 has a higher condition number than Cases 1 and 2, meaning the propagation direction has some impact on crosstalk, but it is insignificant.
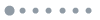
Figure 2.Condition numbers of the MIMO channel matrix in different cases as a function of the RF carrier frequency.
Then, we evaluated the crosstalk impact on the error vector magnitude (EVM) performance and the dynamic range of the RoF link. Figures 3(a) and 3(b) show the comparative EVM performances of different cases versus the RF input power to each RoF link. Note that we forced the input power to the same six RoF links in the test. The EVM value at each point is the root-mean-square (RMS) average of the three streams in Cores 1, 5, and 6. The measurement was carried out at 2.4 GHz, which is one of frequency bands for WLAN. Note that the 2.4 GHz band is not allocated for IEEE 802.11ac in practice. We chose the 2.4 GHz band instead of the 5 GHz band in the measurements, since our RoF transceivers were optimized for the 2.4 GHz band. From the EVM curves, it can be noticed that the crosstalk impact on MCS8 is slightly stronger than that on MCS7. This is mainly because 256QAM, as a higher-order modulation format, is more sensitive to signal-noise radio degradation and thereby more sensitive to crosstalk. Figure 3 further verifies that the propagation direction has some impact on the crosstalk, but it is insignificant. The required EVM for IEEE 802.11ac MSC7 is (4.5%), and it is (3.16%) for MSC8. Given the above EVM threshold, we can observe link dynamic ranges of 28 dB for MSC7 and 23 dB for MSC8. Several typical constellations are also inserted into Figs. 3(a) and 3(b) for reference.
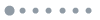
Figure 3.Measured EVM versus RF input power and typical constellations at 2.4 GHz for (a) MCS7 and (b) MCS8.
Setup 2: Fiber + wireless transmission
To verify the feasibility of using MCF instead of a bundle of SMFs, we further built up the 1-m wireless environment in a large room (see Fig. 1). In a mixed fiber-wireless channel, 25 dB-gain power amplifiers (PAs) and 25 dB-gain low-noise amplifiers (LNAs) were used for the downlink and uplink, respectively. In order to keep the wireless environment as stable as possible, we avoided the commercial 2.4 and 5 GHz bands and chose 2.7 GHz as the testing frequency.
Figure 4 shows the average (RMS) EVM performance versus the optical loss of the fiber link. Six optical attenuators whose insert loss are were inserted before the photodiode to vary the optical power input to the receivers. From Fig. 4, we can see the required received optical power is for the uplink and for the downlink for MSC8, given the EVM threshold defined by IEEE 802.11ac. Similarly, optical power is required for MSC7. The lower sensitivity for MSC8 as compared to MSC7 is mainly because the higher modulation format is more sensitive to noise and crosstalk. Moreover, it is also noticed that the sensitivity for the uplink is smaller than that for the downlink. This is mainly because the overall noise figures of the established uplink and downlink are different.
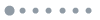
Figure 4.Measured EVM versus optical loss at 2.7 GHz.
Figure 5 shows the condition number of the MSC7 downlink transmission in the 2.66–2.74 GHz bandwidth with different optical losses. The six curves tend to roughly agree with each other, meaning the wireless channel is much more unstable than the fiber channel, and the condition numbers are mainly decided by the wireless channel quality. Similar trends can be found in the MSC7 uplink and the MSC8 uplink and downlink.
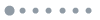
Figure 5.Condition number of cascaded fiber and wireless MIMO channel matrix as a function of the frequency ().
Scenario 2: Full-duplexMIMO transmission
The PolMux method is an effective technique to double the spectral efficiency by transmitting data in two orthogonal polarization modes with the same spectral range. Employing PolMux with MIMO provides a new dimension to double the transmission capacity. Hence, the 7-core fiber can be extended further to achieve a duplex MIMO transmission system. The experimental setup for the PolMux-MIMO downlink is shown in Fig. 6. Every two MIMO signals are polarization multiplexed at a polarization beam combiner. After 2 km MCF transmission, the received signals are demultiplexed by a polarization beam splitter (PBS). The same processes occur in the opposite direction in the uplink; these are not shown in the Fig. 6. The other setups are the same as in Fig. 1. For comparison, MIMO signals are transmitted in the normal MCF in the same propagation direction.
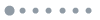
Figure 6.Experimental setup for IEEE 802.11ac-compliant MIMO with PolMux.
Figures 7(a) and 7(b) show the EVM performances for MCS7 and MCS8, respectively, in the MIMO system and the MCF system. We can observe that the EVM performance slightly decreases, especially when the transmitting power is low. This is mainly because that the crosstalk impact is stronger at low power levels. Figure 7(a) depicts the EVM performance of the MCS7 signals. The results show a link dynamic range of 26 dB for the MIMO MCF link and 23 dB for the MIMO link. Similarly, we also evaluated the EVM performance for the MCS8 signals. From Fig. 7(b), we can observe a link dynamic range of 20 dB for the MIMO MCF link and 17 dB for the MIMO link. Above all, employing the PolMux method to double single MCF core usage can greatly increase the system capacity, although it sacrifices the 3 dB dynamic range compared to the non-PolMux MCF links for MCS7 and MCS8.
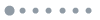
Figure 7.Measured EVM of MIMO systems versus RF input power at 2.4 GHz for (a) MCS7 and (b) MCS8.
Figure 8 shows the condition number value against the RF carrier frequency at 0 dBm RF input power. Within the range from 0–6 GHz, the condition number curve for the MIMO system is higher than that for the MCF MIMO system, which indicates a worse MIMO transmission matrix is measured when using the PolMux method. However, 20 dB (10) is the reference value for the MIMO condition number. The average value of the condition number in the MIMO system from 0 to 6 GHz is 3.78, which is much lower than 10. In summary, although the method will slightly decrease the EVM and condition number performance as opposed to a non-PolMux MCF system, a doubled RF frequency still has many benefits and can improve the system capacity at a low cost.
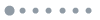
Figure 8.Condition number of MIMO channel matrix as a function of the RF carrier frequency within 6 GHz.
In this Letter, full-duplex transmissions of IEEE 802.11ac-compliant MIMO with wireless transmission and MIMO with PolMux technology are experimentally demonstrated over 2 km MCF and evaluated for in-building WLAN DASs. The results show that the impact of crosstalk on the RoF link performance is not significant. The quality of the cascaded MCF and wireless channel is mainly determined by the wireless part. The method can double the RF frequency efficiency with slight EVM and condition number performance decreases. However, it is still a competitive scheme to improve the system capacity at a low cost.
References
[1] C. Liu, N. Cvijetic, K. Sundaresan, M. Jiang, S. Rangarajan, T. Wang, G. K. Chang. Proceedings of OFC/NFOEC(2013).
[2] Z. Gong, K. Xu, X. Meng, Y. Pei, X. Sun, Y. Dai, Y. Ji, J. Lin. Chin. Opt. Lett., 11, 020603(2013).
[3] K. Xu, R. X. Wang, Y. T. Dai, F. F. Yin, J. Q. Li, Y. F. Ji, J. T. Lin. Photon. Res., 2, 97(2014).
[4]
[5] K. Saitoh, S. Matsuo. Proceedings of OFC/NFOEC, Tutorial Review(2015).
[6] R. Zhu, Y. Zhao, H. Yang, H. Chen, J. Zhang, J. P. Jue. Chin. Opt. Lett., 14, 100604(2016).
[7] M. Morant, R. Llorente, J. Prat. Proceedings of OFC/NFOEC(2014).
[8] M. Morant, A. Macho, R. Llorente. J. Lightwave Technol., 34, 676(2016).
[9] J. Xiao, C. Tang, X. Li, J. Yu, X. Huang, C. Yang, N. Chi. Chin. Opt. Lett., 12, 050603(2014).
[10] S. Alavi, I. Amiri, H. Ahmad, A. Supa’at, N. Fisal. Appl. Opt., 53, 8049(2014).
[11] X. H. Ma, J. Q. Li, S. L. Wang, N. X. Gong, F. F. Yin, Y. T. Dai, K. Xu. Proceedings of ICOCN(2015).
[12] B. Li, X. Ma, J. Sun, H. Zhang, Y. Yao. Opt. Express, 23, 9(2015).
[13] G. S. D. Gordon, M. J. Crisp, R. V. Penty, T. D. Wilkinson, I. H. White. J. Lightwave Technol., 32, 3521(2014).