Abstract
The TiO2 with nanoparticles (NPs), nanowires (NWs), nanorods (NRs) and nanotubes (NTs) structures were prepared by using a in-situ hydrothermal technique, and then proposed as a photoanode for flexible dye-sensitized solar cell (FDSSC). The influences of the morphology of TiO2 on the photovoltaic performances of FDSSCs were investigated. Under rear illumination of 100 mW·cm?2, the power conversion efficiencies of FDSSCs achieved 6.96%, 7.36%, 7.65%, and 7.83% with the TiO2 photoanodes of NPs, NWs, NRs, and NTs and PEDOT counter electrode. The FDSSCs based on TiO2 NRs and NTs photoanodes have higher short circuit current densities and power conversion efficiencies than that of the others. The enhanced power conversion efficiency is responsible for their nanotubes and rod-shaped ordered structures, which are more beneficial to transmission of electron and hole in semiconductor compared to the TiO2 nanoparticles and nanowires disordered structure.Introduction
Dye-sensitized solar cells (DSSCs) with economy, environmental friendliness, mechanical stability, simple preparation process, and high efficiency of 14%, are considered as a favorable choice compared to the silicon device[1−3]. As an important component of DSSC, photoanode is not only a support and adsorption carrier for dye molecules, but also an electron transport carrier. Titanium dioxide (TiO2) is the most representative for its rich optical, dielectric, catalytic, and antimicrobial properties, which leads to a variety of uses in solar cells, photocatalytic, and fuel cells[4−7]. Among three polymorphs of rutile, anatase, and brookite, rutile TiO2 has more stable physicochemical properties, higher refractive index and light-scattering capability compared to anatase[8−10]. Also, the morphology of TiO2 shows significant effect on its physical and chemical properties, such as the one-dimensional (1-D) structure, whose charge transport is preferred compare to the mesoporous structure due to the reduction in grain boundaries and lattice imperfections, thereby slowing down the electron hole recombination rate and accelerating electron transfer, and helping to improve the performance of various optoelectronic applications[11−13]. Sadhu et al. reported the fabrication of a three-dimensional (3-D) rutile-phase TiO2 microsphere arrays on fluorine-doped conducting oxide (FTO), and showed superior photon-harvesting performance owing to the increase in light-scattering in DSSC[14]. Ri et al. spotlighted growth of a sea urchin-like rutile TiO2 hierarchical microsphere on Ti foil, which showed much better light-scattering capability in visible region than the bare Ti foil[15]. Sun et al. recorded a bilayer DSSC with an efficiency of 7.2% based on a bilayer TiO2 film consisting of a 1-D nanowire arrays as underlayer and a 3-D dendritic microsphere as light-scattering overlayer[16]. These results indicate that designing DSSC photoanodes with different morphologies is of great significance for improving the performance of DSSC.
Moreover, the substrate of traditional DSSC is FTO, and its features of rigid and heavy can not meet all application scenarios[17]. To solve this issue, some flexible substrates (e.g., ITO/PET, ITO/PEN and metals) are used to replace FTO glass. Nevertheless, the ITO/PET and ITO/PEN substrates are high-cost and intolerant of temperatures higher than 150 °C, which is much lower than the temperature required for manufacturing photoanodes on FTO glass (450−500 °C), thus resulting in the relatively poor performance of plastic-based DSSCs. Therefore, high-temperature resistant metal foils (such as Ti and stainless steel) are used to fabricate flexible DSSCs (FDSSCs) with encouraging performance compared with the plastic-based ones[18, 19].
In this contribution, the anatase phase TiO2 with four morphologies of nanoparticles, nanowires, nanorods and nanotubes (abbreviated as NP, NW, NR and NT) were prepared by using a in-situ hydrothermal synthesis and served as photoanode in FDSSCs. The influences of the morphology of TiO2 on the adsorption capacity of dyes and charge transfer performances of FDSSCs were extensively investigated. The power conversion efficiencies of the FDSSCs based on NP, NW, NR, and NT TiO2 photoanodes and poly (3,4-ethylenedioxythiophene) (PEDOT)counter electrode reached 6.96%, 7.36%, 7.65%, and 7.83%, respectively under rear illumination of 100 mW·cm−2 and the optimized condition.
Experimental
Various TiO2 photoanode preparation
A reserved thin TiO2 blocking layer was prepared by immersing the Ti substrate in 0.15 M of TiCl4 isopropanol solution for 12 h, followed by sintering at 450 °C for 30 min in air.
Next, four TiO2 photoanodes with different morphologies were prepared.
(1) TiO2 NPs paste were prepared as described previously[20−22].
(2) TiO2 NRs were prepared as following[23−25]. 5 mL Tetrabutyltitanate was rapidly added to 75 mL absolute ethyl alcohol under stirring for 0.5 h and followed by addition of 0.33 mL concentrated sulfuric acid and 0.3 mL deionized water to the solution, and then transferred into 100 mL Teflon-liner and autoclaved at 180 °C for 4 h to form a milky white slurry. The slurry was dissolved into 50 mL, 10 M NaOH solution. After ultrasonication and stirring for 0.5 h until a homogeneous solution was achieved and then the obtained solution was transferred into Teflon-liner and autoclaved at 150 °C for 24 h. The precipitate was collected by filter and washed with distilled water and 0.3 M HCl for 3 times, followed by washing with distilled water some times and annealing at 450 °C for 1 h.
(3) TiO2 NTs were prepared in a two-steps hydrothermal method[26, 27]. 3 g P25 powder was added into 10 M NaOH solution and then transferred into 100 mL Teflon-liner and autoclaved at 160 °C for 4 h and 110 °C for 20 h, respectively. After that, the obtained produce was washed with distilled water and HCl aqueous solution (pH = 2), and then with distilled water for several times until the pH is 7. The products were calcined at 400 °C in air for 1 h. Thus the TiO2 NTs were obtained.
The TiO2 NRs and NTs paste were prepared including of 10 mL absolute ethyl alcohol, 5 mL N-methyl-2-pyrrolidone, 0.1 g polyvinylidene fluoride, 1 g TiO2 NRs and NTs kept stirring for 12 h at 50 °C. Soon afterwards, the TiO2 NPs, NRs, and NTs films were coated onto the preprepared blocking layer by using doctor blade method, and then sintered at 450 °C for 30 min in air.
(4) TiO2 NWs photoanode were prepared as the reported[28−30]. 10 mL dioxane and 1 mL HCl with concentrated of 35% were mixed with stirring, and then added 1 mL titanium butoxide and TiCl4 to above solution. The obtained solution was transferred into a 25 mL Teflon-liner. The Ti substrate with a thin TiO2 blocking layer was placed into above solution at an angle against the wall, and the hydrothermal synthesis was conducted at 180 °C for 6 h. The TiO2 NWs photoanode was obtained.
At last, the dye was loaded by immersing the bilayer TiO2 photoanodes in 0.3 mM of N719 ethanol solution for 12 h. Thus the flexible dye-sensitized TiO2 photoanodes with thickness of 4−5 μm was obtained.
Fabrication of FDSSCs
The PEDOT counter electrode (CE) was electropolymerization from the base polymerization solution consisted of 0.1 M EDOT, 0.1 M LiClO4 and PEG-600 dissolved in anhydrous ethanol. The FDSSCs assembled by clipping the dye-sensitized TiO2 photoanode and Pt CE, and the liquid electrolyte consisted of 0.05 M of iodine, 0.1 M of lituium iodide, 0.6 M of tetrabutylammonium iodide and 0.5 M of 4-tert-butyl-pyridine in acetonitrile was injected in the aperture between the two electrodes.
Characterization
The morphology, microstructure and the elements of the samples were investigated by the transmission electron microscopy (TEM, JEM-2100F, Japan) equipped with energy dispersive spectrometer (EDS) and the field emission scanning electron microscopy (SEM, JSM-7001F, Japan). The crystalline structures of the composites were investigated by glancing incident X-ray diffractometer (X‘Pert Pro, PANalytical B.V., Netherlands). The photovoltaic tests of FDSSCs with an exposed area of 0.2 cm2 were carried out by measuring photocurrent−photovoltage (J−V) characteristic curves under rear irradiation of 100 mW·cm−2 from the solar simulator (CEL-S500, Beijing China Education Au-light Co., Ltd) in ambient atmosphere.
Results and discussions
Fig. 1 shows the SEM images of the TiO2 NPs, NWs, NRs, and NTs. Figs. 1(a)-1 and 1(a)-2 present the porous and loose nanostructure of TiO2 NPs, and the Figs. 1(b)-1 and 1(b)-2 exhibit a mixture of TiO2 NWs and NPs, which are beneficial for the charge transfer and electrolyte loading. To compare the effect of the TiO2 morphology on electron transfer, TiO2 NRs and NTs were prepared and as shown as in Figs. 1(c) and 1(d). From Figs. 1(c)-1 and 1(c)-2, the TiO2 NRs with different sizes and lengths can be observed clearly. Compared to TiO2 NRs, TiO2 NTs with a similar size and length are shown in Figs. 1(d)-1 and 1(d)-2. In a word, the TiO2 photoanode with nano-structure provides highly effective contact between the CE and the I–/I3– redox couple electrolyte, thus possibly absorbing more liquid electrolyte and improving the penetration of I−/I3− liquid electrolyte within TiO2 film, and expecting to obtain an excellent performance for FDSSC.
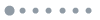
Figure 1.SEM images of TiO2 (a) NPs, (b) NWs, (c) NRs, and (d) NTs.
Table Infomation Is Not EnableTable Infomation Is Not Enable
Fig. 2 displays the XRD patterns of the TiO2 NPs, NWs, NRs, and NTs photoanode. From Fig. 2, the characteristic peaks for the TiO2 NPs (2 = 25.3°, 37.8°, 48.0°, 53.8°, 55.1°, and 62.6°), TiO2 NWs (2 = 25.5°, 38.1°, 48.3°, 54.2°, 55.3°, and 63.0°), TiO2 NRs (2 = 25.7°, 38.4°, 48.7°, 54.3°, 55.5°, and 63.2°), and TiO2 NTs (2 = 26.1°, 38.6°, 48.8°, 54.7°, 55.9°, and 63.5°) associated with the anatase phase are observed in all the XRD patterns[29, 30]. These findings demonstrate that the TiO2 NPs, NWs, NRs, and NTs we synthesized are titania anatase dioxide.
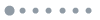
Figure 2.(Color online) XRD patterns of TiO2 NPs, NWs, NRs, and NTs.
Fig. 3 and Table 1 show the N2 adsorption isotherm of the the TiO2 NPs, NWs, NRs, and NTs and the specific surface area are about 91.9122, 116.6744, 126.3625, and 158.8077 m2/g, respectively. The large specific surface area for the TiO2 NRs and NTs, which may be the key factors to absorb more dyes and enhance the performance for FDSSC.
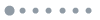
Figure 3.(Color online) Absorption–desorption isotherm of the TiO2 (a) NTs, (b) NRs, (c) NWs, and (d) NPs.
To investigate the effect of TiO2 morphological changes on dye 719 adsorption capacity, Fig. 4 demonstrates the Ultraviolet (UV)–visible absorption spectra of dye N719 made from the flexible TiO2 photoanode with different morphologies, which shows three absorption peaks at 308, 373, and 503 nm for the dye N719. The TiO2 NTs photoanode has the strongest signals, and the orders of the signal strength for the nano TiO2 are NTs﹥NRs﹥NWs﹥NPs. This result indicates that the TiO2 NTs photoanode loads more dyes according to the Lambert–Beer’s law, which means more incident light harvested and the larger photocurrent occurs[31].
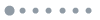
Figure 4.(Color online) The UV−visible absorption spectra of dye desorbed from TiO2 films of NPs, NWs, NRs, and NTs.
Figs. 5(a) and 5(b) are the HRTEM images of TiO2 NTs with approximate lattice spacing of 2.44−3.71 nms (Fig. 5(c)) and mooth surface, which indicates that most of the P25 particles have been changed into TiO2 NTs. The length of the TiO2 NTs is about 100 nm and the diameter is around 15 nm. Fig. 5(d) shows the SAED image of TiO2 NTs. It can be seen that the TiO2 NTs are multicrystal structure, and belongs to the (101), (004), and (200) crystal faces for anatase phase.
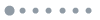
Figure 5.(a-c) HRTEM images and (d) SAED pattern of the TiO2 NTs.
Figs. 6(a) and 6(b) show the SEM images of the porous PEDOT CE. The PEDOT film with uniform network structure is in favour of improving I−/I3− electrolyte’ penetration and enhancing the contact area between CE and electrolyte. Fig. 6(c) shows the CVs of the Pt and PEDOT CEs at the scan rate of 50 mV·s−1. The PEDOT CE have a higher current density (Ipc) absolute value and smaller overpotential (Vpc) than that of the Pt CE. Both the max anode peak current density (Ipa) and Ipc are almost unchanged in Fig. 6(d) which indicates a great electrochemical stability for the PEDOT CE[32]. This phenomenon signifies that the PEDOT CE possesses the Pt-like electrocatalytic ability for I3− reduction due to the low overpotential loss and highly conductive for the PEDOT CE[33].
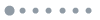
Figure 6.(Color online) (a, b) SEM images of the porous PEDOT CE; (c) the CVs for the Pt and PEDOT CEs; and (d) the 50 successive CV cycles of the PEDOT CE at the scan rate of 50 mV·s−1.
Fig. 7 shows the photovoltaic properties of various FDSSCs under the rear illumination of 100 mW·cm−2 and the detailed parameters are summarized in Table 2. The power conversion efficiencies (PCEs) of the FDSSCs assembled with TiO2 NTs and the Pt and PEDOT CEs in Fig. 7(a) achieves 5.06% and 7.83%, respectively, which is remarkably higher for the latter than that of the former. This is mainly because the much higher transmittance for the PEDOT CE than that of the Pt CE, and the decrease in incident light intensity via the reflection of Pt CE[34, 35]. Fig. 7(b) shows the J−V curves of the FDSSCs based on the PEDOT CE and the TiO2 NPs, NWs, NRs, and NTs photoanodes under the rear irradiation. The FDSSCs with the TiO2 NRs and NTs photoanodes show as high as PCEs of 7.65% and 7.83%, higher than that of the TiO2 NPs (6.96%) and NWs (7.36%) photoanodes. The distinction in performance of the cells possibly is derived from the differences in electronic transmission of nano TiO2. The TiO2 NRs and NTs with directional structure are more conducive to electronic transmission than that of the TiO2 NPs and NWs. Simultaneously, the reduction of dye loading capacity on TiO2 photoanode also plays a certain role for the TiO2 NPs and NWs.
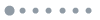
Figure 7.(Color online) J−V characteristics of the FDSSCs fabricated with different photoanodes under the standard illumination.
Fig. 8 presents the Nyqyist plots of the devices with TiO2 NPs, NWs, NRs, and NTs photoanodes and PEDCT CE, in which the performance of devices is directly related to the charge transfer resistance (Rct1 and Rct2)[36]. Because of TiO2 directional structure could enhance the electron collection efficiency in devices, therefore, the cell based on TiO2 NTs photoanode shows the lowest values Rct1 of 2.80 Ω·cm2 and Rct2 of 6.61 Ω·cm2, and the other three devices with the TiO2 NPs, NWs, and NRs photoanodes display the Rct1 of 5.06, 4.17, and 3.59 Ω·cm2, and corresponding to the Rct2 of 10.09, 9.56, and 8.31 Ω·cm2. The former shows the smallest Rct1 and Rct2 compared to the latter cells. This implies that the TiO2 NRs and NTs with directional structure for the devices are better for electronic transmission than that of the TiO2 NPs and NWs, resulting in a higher fill factor (FF) and short-circuit current density. This is in consistent with the results of UV−visible and J−V photovoltaic performance.
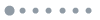
Figure 8.(Color online) EIS spectra of FDSSCs with the various photoanodes and the relevant equivalent circuit model.
Fig. 9 shows the architecture of a FDSSC with TiO2 NTs photoanode irradiated from the rear. The function of the TiO2 blacking layer can reduce the backwash of elections from Ti foil substrate to the electrolyte, thereby enhancing the long-term stability of FDSSC. The TiO2 NTs provide an unobstructed path for electron transfer, which helps improve the efficiency of charge transfer and electron collection. Moreover, the light emitted from the back can penetrate and be absorbed by the N719 dye very well due to the good transparency of the PEDOT CE, producing more photogenerated charge carriers in FDSSC, and resulting greatly enhanced the short-circuit current density and a superior PCE of 7.83%.
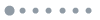
Figure 9.(Color online) Schematic for a FDSSC based on TiO2 NTs photoanode irradiated from the rear.
Conclusions
An efficient FDSSCs assembled with net-work structure’ PEDOT CE and TiO2 NPs, NWs, NRs, and NTs photoanodes achieved power conversion efficiency of 6.96%, 7.36%, 7.65%, and 7.83% under rear illumination of 100 mW·cm−2. The morphology of TiO2 has a significant influence on the photovoltaic performances of FDSSCs. These four FDSSCs with various TiO2 photoanodes morphology possess good fill factor and open circuit voltage, especially for the FDSSCs with TiO2 NRs and NTs photoanodes, which generate higher short circuit current density. This is responsible for their oriented structure of NRs and NTs, which are more beneficial to electron and hole transmission in the semiconductor compared to the TiO2 NPs and NWs photoanodes. This strategy can be further improved by designing a better light-splitting device with vertical incidence and applied other devices with transparent CEs.
Acknowledgements
The authors are very grateful to the joint support by NSFC (No. 61704047). This work is also supported by Science and Technology Development Project of Henan Province (Nos. 212102210126 and 202300410057).
References
[1] J Briscoe, S Dunn. The future of using earth-abundant elements in counter electrodes for dye-sensitized solar cells. Adv Mater Deerfield Beach Fla, 28, 3802(2016).
[2] D O Bellisario, J A Paulson, R D Braatz et al. An analytical solution for exciton generation, reaction, and diffusion in nanotube and nanowire-based solar cells. J Phys Chem Lett, 7, 2683(2016).
[3] K Kakiage, Y Aoyama, T Yano et al. Highly-efficient dye-sensitized solar cells with collaborative sensitization by silyl-anchor and carboxy-anchor dyes. Chem Commun, 51, 15894(2015).
[4] J Y Huang, S H He, W Z Zhang et al. Efficient and stable all-inorganic CsPbIBr2 perovskite solar cells enabled by dynamic vacuum-assisted low-temperature engineering. Sol RRL, 6, 2100839(2022).
[5] A Fujishima, K Honda. Electrochemical photolysis of water at a semiconductor electrode. Nature, 238, 37(1972).
[6] E Hosono, H Matsuda, I Honma et al. Synthesis of a perpendicular TiO2 nanosheet film with the superhydrophilic property without UV irradiation. Langmuir, 23, 7447(2007).
[7] Z T Han, S S Li, J J Li et al. Facile synthesis of ZnO nanowires on FTO glass for dye-sensitized solar cells. J Semicond, 34, 074002(2013).
[8] D L Gapale, P P Bardapurkar, S A Arote et al. Humidity sensing properties of spray deposited Fe doped TiO2 thin film. J Semicond, 42, 122805(2021).
[9] A G Thomas, K L Syres. Adsorption of organic molecules on rutile TiO2 and anatase TiO2 single crystal surfaces. Chem Soc Rev, 41, 4207(2012).
[10] X F Cai, P Zhang, S H Wei. Revisit of the band gaps of rutile SnO2 and TiO2: A first-principles study. J Semicond, 40, 092101(2019).
[11] X J Feng, K Shankar, O K Varghese et al. Vertically aligned single crystal TiO2 nanowire arrays grown directly on transparent conducting oxide coated glass: Synthesis details and applications. Nano Lett, 8, 3781(2008).
[12] B Liu, E S Aydil. Growth of oriented single-crystalline rutile TiO2 nanorods on transparent conducting substrates for dye-sensitized solar cells. J Am Chem Soc, 131, 3985(2009).
[13] S Sadhu, A Jaiswal, S Adyanthaya et al. Surface chemistry and growth mechanism of highly oriented, single crystalline TiO2 nanorods on transparent conducting oxide coated glass substrates. RSC Adv, 3, 1933(2013).
[14] S Sadhu, P Gupta, P Poddar. Physical mechanism behind enhanced photoelectrochemical and photocatalytic properties of superhydrophilic assemblies of 3D-TiO2 microspheres with arrays of oriented, single-crystalline TiO2 nanowires as building blocks deposited on fluorine-doped tin oxide. ACS Appl Mater Interfaces, 9, 11202(2017).
[15] J H Ri, S F Wu, J P Jin et al. Growth of a sea urchin-like rutile TiO2 hierarchical microsphere film on Ti foil for a quasi-solid-state dye-sensitized solar cell. Nanoscale, 9, 18498(2017).
[16] S H He, L W Shang, Y Y Gao et al. Holistically modulating charge recombination via trisiloxane surface treatment for efficient dye-sensitized solar cells. J Alloys Compd, 896, 162864(2022).
[17] K Fan, R J Li, J N Chen et al. Recent development of dye-sensitized solar cells based on flexible substrates. Sci Adv Mat, 5, 1596(2013).
[18] L X Song, X Yin, X Y Xie et al. Highly flexible TiO2/C nanofibrous film for flexible dye-sensitized solar cells as a platinum- and transparent conducting oxide-free flexible counter electrode. Electrochim Acta, 255, 256(2017).
[19] S H He, Z Lan, B Zhang et al. Holistically optimizing charge carrier dynamics enables high-performance dye-sensitized solar cells and photodetectors. ACS Appl Mater Interfaces, 14, 43576(2022).
[20] X M Gao, Z T Shen, G T Yue et al. Sodium molybdate-assisted synthesis of a cobalt phosphide hybrid counter electrode for highly efficient dye-sensitized solar cells. ACS Appl Energy Mater, 4, 3851(2021).
[21] Y Du, Z Shen, G Yue et al. CoP@Ni2P microcrystals in situ grown on carbon fiber as counter electrode catalysts for high-efficiency dye-sensitized solar cells. Mater Today Sustain, 20, 100262(2022).
[22] Y He, Z T Shen, G T Yue et al. A dye-sensitized solar cells with enhanced efficiency based on a “pillared effect” of CoMoP2 @Mxene@CNTs composite counter electrode. J Alloys Compd, 922, 166279(2022).
[23] M Gao, Z T Shen, G T Yue et al. One-pot hydrothermal in situ growth of In4SnS8@MoS2@CNTs as efficient Pt-free counter electrodes for dye-sensitized solar cells. J Alloys Compd, 932, 167643(2023).
[24] F D Yu, G S Han, Y J Tu et al. Electron extraction mechanism in low hysteresis perovskite solar cells using single crystal TiO2 nanorods. Sol Energy, 167, 251(2018).
[25] Z Lan, X X Xu, X Z Zhang et al. Low-temperature solution-processed efficient electron-transporting layers based on BF4−-capped TiO2 nanorods for high-performance planar perovskite solar cells. J Mater Chem C, 6, 334(2018).
[26] W C Chen, M H Yeh, L Y Lin et al. Double-wall TiO2 nanotubes for dye-sensitized solar cells: A study of growth mechanism. ACS Sustainable Chem Eng, 6, 3907(2018).
[27] M Guo, J Chen, J Zhang et al. Coupling plasmonic nanoparticles with TiO2 nanotube photonic crystals for enhanced dye-sensitized solar cells performance. Electrochim Acta, 263, 373(2018).
[28] R T Ako, P Ekanayake, C M Lim. An analysis of DSSC performance based on nanosphere, nanorod, and nanoparticle anode morphologies. J Appl Phys, 120, 1089(2016).
[29] Y Y Liu, X Y Ye, Q Q An et al. A novel synthesis of the bottom-straight and top-bent dual TiO2 nanowires for dye-sensitized solar cells. Adv Powder Technol, 29, 1455(2018).
[30] Q Q Qiu, S Li, J J Jiang et al. Improved electron transfer between TiO2 and FTO interface by N-doped anatase TiO2 nanowires and its applications in quantum dot-sensitized solar cells. J Phys Chem C, 121, 21560(2017).
[31] Y M Xiao, J H Wu, G T Yue et al. The preparation of titania nanotubes and its application in flexible dye-sensitized solar cells. Electrochim Acta, 55, 4573(2010).
[32] K X Li, G T Yue, F R Tan. A binder-free CF|PANI composite electrode with excellent capacitance for asymmetric supercapacitors. J Semicond, 44, 032701(2023).
[33] X Q Liu, Y Liang, G T Yue et al. A dual function of high efficiency quasi-solid-state flexible dye-sensitized solar cell based on conductive polymer integrated into poly (acrylic acid-co-carbon nanotubes) gel electrolyte. Sol Energy, 148, 63(2017).
[34] J H Wu, Y Li, Q W Tang et al. Bifacial dye-sensitized solar cells: A strategy to enhance overall efficiency based on transparent polyaniline electrode. Sci Rep, 4, 4028(2014).
[35] Y Du, G T Yue, Z Lan et al. A dye-sensitized solar cell based on magnetic CoP@FeP4@Carbon composite counter electrode generated an efficiency of 9.88%. Inorg Chem Front, 8, 5034(2021).
[36] Q W Tang, W L Zhu, B L He et al. Rapid conversion from carbohydrates to large-scale carbon quantum dots for all-weather solar cells. ACS Nano, 11, 1540(2017).