Author Affiliations
1Department of Physics and Astronomy, San Francisco State University, San Francisco, California 94132, USA2Department of Electrical and Computer Engineering, University of California San Diego, La Jolla, California 92093, USA3Department of NanoEngineering, University of California San Diego, La Jolla, California 92093, USA4Department of Biology, San Francisco State University, San Francisco, California 94132, USA5The MOE Key Laboratory of Weak-Light Nonlinear Photonics, and TEDA Applied Physical Institute and School of Physics, Nankai University, Tianjin 300457, Chinashow less
Abstract
We design and demonstrate new types of optical tweezers with lateral pulling forces that allow full control of biological samples with complex geometric shapes. With appropriate beam shaping, the dual tug-of-war tweezers effectively hold and stretch elongated biological objects of different sizes, and the triangular tug-of-war tweezers with threefold rotational symmetry steadily hold asymmetric objects in the plane of observation and exert stretching forces along three directions. We successfully apply these tweezers to manipulate microparticles and bacterial cells in aqueous media.Since the pioneering work of Ashkin and his co-workers[1], the realm of optical tweezers has expanded tremendously. Optical trapping and manipulation has developed into an interdisciplinary field, influencing subjects as diverse as medicine, soft condensed matter, and atmospheric sciences[2–5]. Many applications require the tweezers to make dynamical optical traps capable of full three-dimensional (3-D) control of the trapped objects.
In microbiology, as pathogens evolve increased resistance to antibiotics[6], new tools, including optical ones, that facilitate the study of bacterial growth, cell-cell interactions, and biofilm formation are highly desired. Among the vast array of bacterial cell morphologies, the rod shape continues to be prevalent in genetic models and its generation and maintenance has been best studied, but there is a renewed appreciation for asymmetric and complex shapes[7–9].
Unfortunately, there are few methods to trap and orient rod-shaped and asymmetric bacteria in the observation plane. A single-beam gradient laser trap is one of the most common techniques for optical trapping, but a rod-shaped bacterium automatically aligns its major axis parallel to the trapping beam as soon as the bacterium is trapped [see Fig. 1(a)]. This automatic alignment of the bacteria deters direct observations of cell structures, motility, and other characteristics.
Sign up for Chinese Optics Letters TOC. Get the latest issue of Chinese Optics Letters delivered right to you!Sign up now
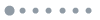
Figure 1.Different designs of optical tweezers. (a) Single-beam optical tweezers align an elongated object along the beam axis. (b) Dual-beam optical tweezers hold an object from each end. (c) TOW optical tweezers trap an object at each end and pull the ends in opposite directions. (d) Triangular TOW tweezers with threefold rotational symmetry allow trapping and stretching of an irregularly shaped object.
Holographic optical tweezers[2–5] have been improved with dual or multiple traps to achieve enhanced control of a large number of particles, along with those created with complex beam shaping techniques[10–14]. Many fields have benefited from the advancement of such tweezers, although they still have some limitations. For instance, when using holographic optical tweezers with two single-beam gradient traps side by side [see Fig. 1(b)], the stability of the trap is delicate, and independent control of the two traps is necessary. Due to Brownian motion and bacterial motility, a bacterium often slips and becomes trapped and flipped in one of the trapping beams.
In this Letter, we present a new approach to achieve stable in-plane optical trapping and full control of rod-shaped and irregularly shaped bacteria. This approach is based on a special design of what we call tug-of-war (TOW) optical tweezers [see Fig. 1(c)][15], which accommodate different bacterial shapes and require less trapping power. Such TOW tweezers dramatically improve the trapping stability. In addition to dynamic control of rod-shaped objects, these optical tweezers can be used simultaneously as an optical stretcher, applying a tunable lateral pulling force to a cell or a cell cluster. Both gradient and scattering forces work together in pulling groups of cells apart[15]. To trap cells or cellular clusters with more complicated shapes, we designed and established triangular TOW optical tweezers [see Fig. 1(d)]. The triangular TOW tweezers allow stretching of cells in three directions, while holding them steadily in the plane of observation. Of course, as we create more lobes in the TOW tweezers, the power of the trap splits and the tweezers require more laser power. These TOW optical tweezers may find a variety of biological applications, such as the study of cellular viscoelasticity, motility, and intercellular interactions.
In designing the dual TOW optical tweezers, instead of using two separate, independently controlled Gaussian traps, we split one beam into a pair of diverging elongated beams. The divergence of the beams creates the lateral pulling force on the rod-shaped object. We designed the beam with a hologram in a spatial light modulator (SLM), creating a virtual cylindrical lens with an adjustable focal length and a prism with an adjustable apex angle [Fig. 2(a)]. By using the Fourier beam propagation method, the 3-D volumetric rendering of the dual TOW beam was constructed from experimental data near the focus of an objective lens, as shown in Media 1 and Figs. 2(b)–2(d). This TOW design, utilizing the opportunities granted by the SLM, leads to a new and effective optical tool for in-plane trapping and control of rod-shaped objects [Figs. 2(e)–2(h)]. By adjusting the diverging angle, the size of the elongated beams, and the laser power, we can optimize our dual TOW tweezers to trap cells of different lengths and different indices of refraction, stretch them, and even break them apart. The TOW tweezers can apply a tunable lateral pulling force on the trapped object with reduced laser power per area and improved stability as compared to the single-beam gradient laser trap[15].
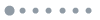
Figure 2.Dual TOW beam: (a) a hologram, (b–d) projections of a volumetric rendering of the dual TOW beam from experimental data onto different planes near the focus of an objective lens, as the beam propagates in the direction (Media 1). (e–h) An illustration of the dynamic process of how a bacterial cluster is trapped, stretched, and separated by the TOW tweezers.
Experimental results using the dual TOW optical tweezers for trapping, translating, and rotating rod-shaped objects are shown in Fig. 3. The simplest demonstration of the TOW mechanism is trapping a silica rod [see Media 2 and Figs. 3(a)–3(d)]. When the TOW tweezers trap a silica rod (6 μm), the rod oscillates near the trap and reorients itself, eventually being trapped by both sides of the TOW beam in the observation plane and held firmly. After trapping, a silica rod can easily be translated or rotated. With a quick change of the parameters in the LabView program controlling the SLM, the size of the dual trap can be optimized for particles of different sizes and materials. To show the potential of the method for biological applications, in Figs. 3(e)–3(l) we demonstrated the use of dual TOW tweezers for trapping and manipulation of different types and sizes of bacteria. Both Escherichia coli (Gram-negative, long) and Bacillus thuringiensis (Gram-positive, long) are trapped and reoriented in the observing plane with reliable stability.
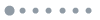
Figure 3.Optical trapping and manipulation of rod-shaped objects with dual TOW tweezers. (a–d) Snapshots from a video (Media 2) showing a silica rod being trapped and rotated. (e–h) Translation and rotation of a 5-μm B. thuringiensis bacterium (Media 3). (i–j) Manipulation of a 2 μm E. coli bacterium. (k–l) Rotation of an 18 μm long B. thuringiensis bacterium. (m–p) Stretching and breaking apart a cluster of S. meliloti cells. Scale bar: 3 μm.
The TOW tweezers are also excellent for stretching bacteria and separating Sinorhizobium meliloti (S. meliloti) bacteria clusters [Figs. 3(m)–3(p)]. S. meliloti is a Gram-negative soil bacterium capable of holding a symbiotic relationship with host plants. It serves as a model for studying microbe–host interactions and has a similar organelle development and cellular differentiation to other bacteria[16,17]. Our experiments show that the pulling force in the TOW tweezers is strong enough to hold a bacterial cluster and even to break it apart[15]. This is not achievable by using dual trap optical tweezers[18] or an optical stretcher formed by two counterpropagating beams[19]. As an example, this stretching ability was applied to study in detail the biofilm strength of S. meliloti aggregated in different growing conditions.
The triangular TOW tweezers utilize the same concept as the dual TOW tweezers. With three lobes instead of two, the triangular TOW tweezers are useful for trapping asymmetric objects as well as objects with threefold rotational symmetry. In designing the triangular TOW tweezers, the SLM hologram creates a virtual three-sided pyramidal lens that splits the beam into three stripes. If the single beam is split only by using a three-sided pyramid, the beam will create three symmetric dots in the focal plane, as shown in simulation in Figs. 4(a) and 4(b) and experimentally in Fig. 4(c). However, by introducing the corresponding combination of virtual cylindrical lenses, the beam will have an additional parabolic trajectory with elongated traps in the focal plane [Figs. 4(d)–4(g)]. This parabolic curvature in the beam creates strong field gradients while at the same time also creating a net outward momentum flux acting away from the center that pushes trapped asymmetric objects outward in all three directions. While in some sense this parabolic trajectory is quite similar to that of the self-accelerating autofocusing beams[20,21], simulation showed that in the far field the beam breaks into three spatially separated triangular shaped beams. In principle, the TOW tweezers may be reconfigured to include dynamical traps[22], or to allow temporal modulation by changing the spatial profile of the tweezers, but we have not developed these possibilities in the current work.
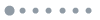
Figure 4.(a–b) Simulation of (a) a hologram and (b) the amplitude in focus of a triangular TOW beam without a parabolic trajectory. (c) The experimental profile of the triangular TOW beam corresponding to (b). (d–f) Corresponding results for a triangular TOW beam with a parabolic trajectory. (g) Volumetric image of the triangular TOW beam calculated via the beam propagation method (Media 4).
Experimental results for trapping and manipulation with triangular TOW tweezers are shown in Fig. 5. There are two easy ways to create experimentally symmetrical intensity profiles with the triangular TOW beam when using a square or rectangular-shaped SLM screen: 1) amplitude modulate the intensity at the plane of the SLM or 2) underfill the SLM with a Gaussian beam to evenly cover each side of the triangle. In Figs. 5(a)–5(c) the triangular TOW tweezers trapped three silica spheres of diameter 2 μm with one sphere stably trapped by each lobe of the tweezers. As the spheres are trapped, they may be rotated and moved radially inward and outward in the observation plane by reconfiguring the SLM setting.
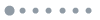
Figure 5.Optical trapping and manipulation of objects with triangular TOW tweezers. (a–c) Trapping, rotating, and translating 3 μm polystyrene beads (Media 5). (d–f) Trapping and rotating a mutant multipronged S. meliloti bacterial cell. Scale bar: 5 μm.
To determine how efficiently triangular TOW tweezers can trap asymmetric objects, we tested them on more complicated morphologies, which can occur in rod-shaped cells due to genetic mutations, physical clustering, or environmental factors. Here we demonstrated trapping and manipulation of irregularly shaped, multipronged bacterial cells produced by a mutant strain of S. meliloti in lysogeny broth (LB) media [Figs. 5(d)–5(f)]. While S. meliloti normally generate rod-shaped cells, a deletion of the polarity-determinant gene podJ1 causes the mutant cells to form multipronged clumps[15]. To capture and provide dynamic control over translation and rotation of trapped bacteria, the size of the triangular TOW tweezers can be adjusted to match bacterial morphology.
The ability to stably trap rod-shaped and asymmetrically shaped specimens creates new opportunities for studying the differences between healthy and diseased cells, particularly mammalian ones, in which changes in biomechanics are strongly linked to disease states[23]. With dual optical traps, researchers found subtle differences in the biomechanical properties of red blood cells associated with diabetes[24,25]. Also, cancer cells often have a larger Young’s modulus than healthy cells[26], and optical tweezers may be useful for analyzing their mechanical properties. The ability of TOW tweezers to provide tunable lateral pulling forces may offer enhanced function and capability for these studies compared to the conventional optical traps.
In addition to studying biological samples, optical tweezers have been used innovatively to engineer nanostructures. For example, 3-D structures were recently constructed from microtubules[27] and semiconductor nanowires[28], demonstrating significant advances in nanoengineering. TOW tweezers may be useful in similarly delicate processes of synthesizing nanostructures due to their unusual capability for dynamic control.
In conclusion, we believe our technique brings about many exciting possibilities. TOW tweezers can be quickly implemented and precisely controlled as a new tool that may find various nanotechnology and biomedical applications.
References
[1] A. Ashkin, J. M. Dziedzic. Science, 235, 1517(1987).
[2] D. G. Grier. Nature, 424, 810(2003).
[3] D. McGloin, J. P. Reid. Opt. Photonics News, 21, 20(2010).
[4] M. Padgett, R. Bowman. Nat. Photonics, 5, 343(2011).
[5] K. Dholakia, T. Čižmár. Nat. Photonics, 5, 335(2011).
[6] . Antimicrobial Resistance: Global Report on Surveillance(2014).
[7] K. D. Young. Microbiol. Mol. Biol. Rev., 70, 660(2006).
[8] A. M. Randich, Y. V. Brun. Front. Microbiol., 6, 580(2015).
[9] D. T. Kysela, A. M. Randich, P. D. Caccamo, Y. V. Brun. PLoS Biol., 14, e1002565(2016).
[10] R. Agarwal, K. Ladavac, Y. Roichman, G. Yu, C. M. Lieber, D. G. Grier. Opt. Express, 13, 8906(2005).
[11] S. Simpson, S. Hanna. J. Opt. Soc. Am. A., 27, 1255(2010).
[12] F. Hoerner, M. Woerdemann, S. Muller, B. Maier, C. Denz. J. Biophotonics, 3, 468(2010).
[13] P. Zhang, D. Hernandez, D. Cannan, Y. Hu, S. Fardad, S. Huang, J. Chen, D. N. Christodoulides, Z. Chen. Biomed. Opt. Express, 3, 1892(2012).
[14] J. Zhao, I. D. Chremmos, D. Song, D. N. Christodoulides, N. K. Efremidis, Z. Chen. Sci. Rep., 5, 12086(2015).
[15] A. Bezryadina, D. Preece, J. Chen, Z. Chen. Light Sci. Appl., 5, e16158(2016).
[16] A. T. Fields, C. S. Navarrete, A. Z. Zare, Z. Huang, M. Mostafavi, J. C. Lewis, Y. Rezaeihaghighi, B. J. Brezler, S. Ray, A. L. Rizzacasa, M. J. Barnett, S. R. Long, E. J. Chen, J. C. Chen. Mol. Microbiol., 84, 892(2012).
[17] K. M. Jones, H. Kobayashi, B. W. Davies, M. E. Taga, G. C. Walker. Nat. Rev. Microbiol., 5, 619(2007).
[18] E. Fällman, O. Axner. Appl. Opt., 36, 2107(1997).
[19] J. Guck, R. Ananthakrishnan, H. Mahmood, T. J. Moon, C. C. Cunningham, J. Kas. Biophys. J., 81, 767(2001).
[20] P. Zhang, J. Prakash, Z. Zhang, M. S. Mills, N. K. Efremidis, D. N. Christodoulides, Z. Chen. Opt. Lett., 36, 2883(2011).
[21] D. G. Papazoglou, N. K. Efremidis, D. N. Christodoulides, S. Tzortzakis. Opt. Lett., 36, 1842(2011).
[22] L. Ling, H. L. Guo, L. Huang, E. Qu, Z. L. Li, Z. Y. Li. Chin. Phys. Lett., 29, 014214(2012).
[23] S. Subra. Acta Biomater., 3, 413(2007).
[24] R. Agrawal, T. Smart, J. Nobre-Cardoso, C. Richards, R. Bhatnagar, A. Tufail, D. Shima, P. H. Jones, C. Pavesio. Sci. Rep., 6, 15873(2016).
[25] T. J. Smart, C. J. Richards, R. Bhatnagar, C. Pavesio, R. Agrawal, P. H. Jones. Proc. SPIE, 9548, 954825(2015).
[26] M. Lekka, K. Pogoda, J. Gostek, O. Klymenko, S. Prauzner-Bechcicki, J. Wiltowska-Zuber, J. Jaczewska, J. Lekki, Z. Stachura. Micron, 43, 1259(2012).
[27] J. Bergman, O. Osunbayo, M. Vershinin. Sci. Rep., 5, 18085(2015).
[28] O. Maragò, P. Jones, P. Gucciardi, G. Volpe, A. Ferrari. Nat. Nanotechnol., 8, 807(2013).