Author Affiliations
1Department of Electronic Engineering, Xiamen University, Xiamen 361005, China2Jiangsu Key Laboratory of Advanced Laser Materials and Devices, School of Physics and Electronic Engineering, Jiangsu Normal University, Xuzhou 221116, China3School of Physics Science and Engineering, Institute for Advanced Study, Tongji University, Shanghai 200092, China4Department of Physics, Sofia University, 5 James Bourchier Blvd., 1164 Sofia, Bulgaria5e-mail: xdxu79@jsnu.edu.cnshow less
Abstract
We report on a diode-end-pumped high-power and high-energy Nd:YAG single-crystal fiber laser at 1834 nm. Two 808 nm diodes injecting about 58 W pump power into the Nd:YAG fiber have generated 3.28 W continuous-wave and 1.66 W Cr:ZnSe-based passively -switched lasers. Slope efficiencies with respect to pump powers are 8.7% for the continuous-wave laser and 4.9% for the -switched laser. The extracted maximum pulse energy is about 266.9 μJ, and the corresponding maximum pulse peak power is 2.54 kW. These performances greatly surpass previous results regarding this specific laser emission because the laser gain medium in the form of fiber can significantly mitigate thermally induced power saturation thanks to its significantly reduced thermal lensing effect. Single-crystal fiber lasers show great potential for high average power, pulse energy, and peak power.1. INTRODUCTION
Compact and efficient eye-safe laser sources in the 2.0 μm spectral region are attractive for many applications, including laser radar, remote sensing, spectroscopy, medical treatment, optical communications, and metrology [1–3]. It is well known that laser sources in this specific wavelength region can be widely provided by -doped diode-pumped solid-state and fiber lasers. However, it is a quasi-three-level system according to the energy-level structure of ions, which indicates the existence of reabsorption loss. Despite broad emission spectra extending to shorter than 1.8 μm for many laser materials, the efficient lasing wavelengths have still been limited to longer than about 1.9 μm. Choice of laser sources with wavelengths shorter than 1.9 μm could be particularly needed for shallow water absorption of biological tissues [4].
On the other hand, lasers have been commonly developed as laser sources at 0.9, 1.06, 1.1, 1.3, and 1.4 μm, respectively, corresponding to emission transitions from the upper level of to lower levels of , , and . Another emission channel of corresponding to to transition with emission lines at around 1.8 μm has been far less studied because of its small emission cross section ( for Nd:YAG [5]) in comparison with other lines. However, in fact, for Nd:YAG this value is indeed comparable with the emission cross sections of many -doped materials, even higher. For instance, the emission cross sections of Tm:YAG, Tm:GGG, Tm:LuAG, and Tm:YLF are all [6–8]. Moreover, the 1.8 μm lasers belong to a four-level system; therefore, no reabsorption effect exists.
Development toward the realization of high-power 1.8 μm laser sources has been far limited in the past years. Back to 1971, the first laser oscillation at this line was demonstrated by using Kr flash lamp pumping with Nd:YAG cooling down to [9]. In 1992, Kubo and Kane [10] operated a diode-pumped 3 mW 1833 nm Nd:YAG laser, and the crystal was cooled to . Much better laser performance was obtained later by operating Nd:YAG waveguides for improving this laser emission to 0.4 W [11] and 1.4 W [12] by the same group. In 2016, we reported a 1834 nm Nd:YAG bulk laser with a maximum output power of 1.31 W [13]. However, at present, no higher power than 1.4 W has been achieved at this specific laser line, and pulsed laser operation at this line has not yet been reported.
Sign up for Photonics Research TOC. Get the latest issue of Photonics Research delivered right to you!Sign up now
For further improving the 1.8 μm laser output power, simply increasing the pump power is a primary consideration. However, the quantum defect for 808 nm diode pumped 1.8 μm lasers is about 56%, which is a serious negative factor in restricting the laser output power improvement because of the thermal lensing effect. Low dopant concentration is favorable for thermal alleviation and upconversion loss reduction. To ensure sufficient pump power absorption, a long gain medium should be adopted. Based on this idea, a laser gain medium in the form of fiber could be advantageous. Moreover, crystalline fiber shares the advantage of optical fiber with an excellent thermo-optic effect because of the large surface-volume ratio. In fact, recently, [14–18], [19,20], [21], and [22] crystalline-fiber lasers for power scaling have already been demonstrated. For instance, 250 W Nd:YAG [14] and 250 W Yb:YAG [19] single-crystal fiber lasers have been reported with high-power diode lasers as pump sources in longitudinal pumping configuration.
In this research, adopting a Nd:YAG single-crystal fiber as a gain medium, we have realized the highest output power of a 1.8 μm Nd:YAG laser in a continuous-wave regime. We have further operated the 1.8 μm Nd:YAG laser in a passively -switched regime with Cr:ZnSe crystal. It is worthwhile to mention that, apart from the aforementioned various applications, the high-power 1834 nm Nd:YAG laser source could be much more efficient to pump than presently used 1.9 μm lasers [23] for the realizations of tunable and ultrafast middle infrared lasers.
2. EXPERIMENTAL SETUP
Figure 1(a) shows a photograph of the as-prepared Nd:YAG single-crystal fiber with a diameter of 0.8 mm and length of 20 mm, which was grown by the micro-pulling-down (μ-PD) method. The dopant concentration of is 0.2%. The Nd:YAG fiber was antireflection coated at 1.83 μm on two faces. Moreover, it was wrapped with indium foil and mounted inside a copper block. The copper block was connected to a water-cooled chiller with temperature set at 14°C. Figure 1(b) shows the emission spectrum of the Nd:YAG fiber under a 808 nm diode excitation, from which one can clearly see an emission peak at about 1834 nm. Figure 2 shows the laser experimental setup schematically. A double-end pumping geometry was adopted to operate the Nd:YAG fiber laser. In general, double-end pumping benefits the reduction of local temperature increase and improves the distribution of population inversion all over the fiber. The two fiber-coupled diode pump sources have similar parameters with a size of 200 μm and numerical aperture of 0.22. The maximum output powers are actually about 28 and 30 W. The Rayleigh length of the diode laser is about 1.8 mm; therefore, the pump beam is guided through the fiber via total internal reflection.
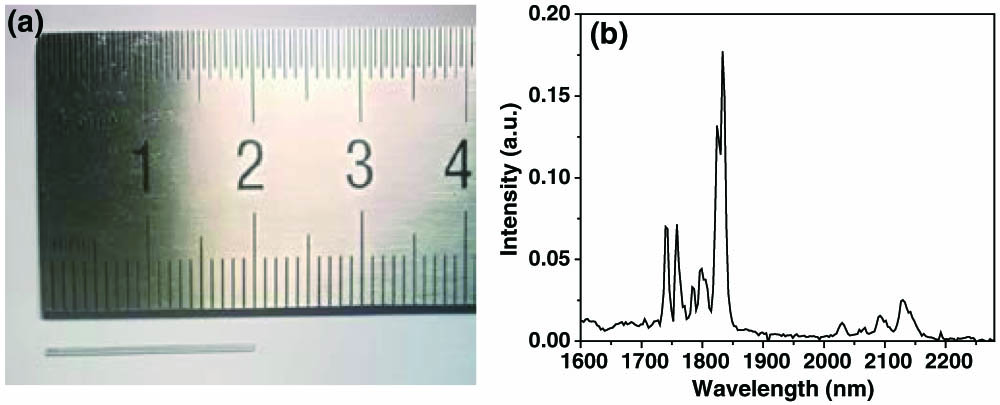
Figure 1.(a) Photograph of the as-grown Nd:YAG single-crystal fiber via the μ-PD technique. (b) Emission spectrum of Nd:YAG single-crystal fiber at around 1.8 μm under 808 nm diode excitation.
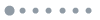
Figure 2.Schematic of the diode-pumped Nd:YAG fiber laser experimental setup.
Single-pass absorption measurement of the Nd:YAG fiber gave an absorption ratio of about 91% of the pump power. Moreover, changing the focusing point of the pump beam inside the crystal fiber, we observed that the absorption ratio was almost exactly the same, which probably indicated that the ions have been doped into the YAG host uniformly. The homogeneous dopant concentration distribution is of benefit to good laser performance. More precise radial and axial concentration distributions in the grown crystal fiber should be measured by electron probe microanalysis, which is not available at the moment.
The left-hand diode laser was collimated and focused by two 50 mm (focal length) doublet lenses into the fiber, while 75 mm doublet lenses were used for the right-hand diode laser. A three-mirror V-type laser resonator was configured with curvature radii of 50, 100, and 50 mm, as shown in Fig. 2. The two input mirrors have high transmission of more than 94% at the pumping wavelength and high reflection of more than 99.9% at 1834 nm. The output coupler has a transmission of about 5.8% at 1834 nm. Additionally, the two input mirrors and output coupler all have high transmissions (more than 80% at least) at those high-gain lines (1.06, 1.1, 1.3, and 1.4 μm) to suppress lasing of these lines. A Cr:ZnSe crystal with an initial transmission of 96% at 1834 nm was used to play the role as a switcher [24]. At this wavelength, the absorption cross section of Cr:ZnSe crystal is about [25], which is far larger than the emission cross section of Nd:YAG at 1834 nm and thus favorable for passive -switching.
3. RESULTS AND DISCUSSION
The crystal structure of Nd:YAG crystal fiber was examined with X-ray diffraction (XRD, Bruker-D2, Germany). The result showed that the Nd:YAG crystal fiber had the same structure as a YAG single crystal. The lattice constant was calculated to be . We also evaluated the optical quality of the Nd:YAG fiber by coupling a nondiffracted (by the edges of the crystal fiber) 1834 nm Nd:YAG bulk laser into the sample. The transmission was measured to be better than 99%, which indicates good optical quality of the fiber sample. The other 1% loss should be mainly attributed to scattering losses. Nevertheless, the relatively low loss can be readily compensated by the high gain available in the crystal fiber.
Continuous-wave laser operation was first fulfilled. Figure 3 shows the output power varying with the increase of pump power. The laser behavior occurred at a threshold of about 7.87 W of pump power. The maximum output power reached 3.28 W at a pump power of approximately 58 W, and the slope efficiency can be fitted to be about 8.7%. The efficiency is a bit smaller than the previous result from a Nd:YAG bulk laser. However, for longitudinal pumping, it is difficult to avoid thermally induced fracture of the gain medium for such a high pump power up to 58 W. For instance, Lee et al. [26] reported a good result in regard to an 808 nm diode-end-pumped composite YAG-Nd:YAG-YAG bulk laser at 1415 nm. The output power showed rollover at an absorbed power of about 28 W. We recently found that the thermally induced output power saturation occurred at an absorbed power of about 23 W for a 1.4 μm laser using a conventional Nd:YAG bulk crystal [27]. Note that the 1.8 μm laser has a large quantum defect indicating a much severer thermal effect for emission at this line. However, at this present experiment, we have not observed obvious rollover of the output power. In addition, we measured the output power fluctuation at maximum to be about 1.1% (rms) in 20 min, as shown in Fig. 3 as an inset, which indicates good thermal stability. The typical laser spectrum is registered in Fig. 4. Using a Bristol Model 721B-IR laser wavelength meter with wavelength resolution of 6 GHz (about 0.06 nm), we accurately measured the laser wavelength to be about 1834.58 nm with a full width at half-maximum (FWHM) of about 0.78 nm.
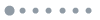
Figure 3.Dependence of output power on pump power of the continuous-wave and -switched Nd:YAG fiber lasers.
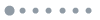
Figure 4.Typical laser spectrum of the Nd:YAG fiber laser at about 1834 nm.
A passively -switched Nd:YAG laser at 1834 nm has, to the best of our knowledge, never been demonstrated. Inserting the Cr:ZnSe crystal into the folded arm of the laser resonator, at a pump power close to 13 W, stable -switched pulse trains were observed. When increasing the pump power to maximum, the -switched Nd:YAG fiber laser reached a maximum average output power of 1.66 W. Linear fitting the data, we found a slope efficiency of about 4.9%. Figure 5 shows the typical pulse trains and single-pulse profiles. Figure 5(a) shows the pulse trains with repetition rates of 1.10 kHz at average output power of 0.11 W (three pulses in 100 μs/div scale). Figure 5(b) shows the corresponding single pulse profile with duration of 130.2 ns (FWHM). Figure 5(c) shows the pulse trains obtained at a maximum output power of 1.66 W. Under the same time scale, 15 pulses were observed, which indicates a repetition rate of 6.34 kHz. Figure 5(d) shows the corresponding pulse width of about 104.6 ns, which is almost the shortest pulse achieved in this work. In fact, according to Fig. 6(a), showing the complete pulse widths varying from the threshold to maximum pump power, one can find that the pulse width, from the initial width of about 146 ns at threshold to the minimum width of about 104 ns at pump power of 45.5 W, showed a saturation effect. Correspondingly, the pulse repetition rate increased from 0.58 to 6.34 kHz with increase of the pump power [see Fig. 6(b)]. As a result, combining the average output power, we can calculate the single-pulse energy and pulse peak power, as shown in Figs. 6(c) and 6(d), respectively. The achieved maximum pulse energy and peak power reached about 266.9 μJ and 2.54 kW. The pulse energy and peak power both showed saturations. One of the reasons that limited the increases of the pulse energy and peak power could be the high-transmission saturable absorber. Note that such laser performance is difficult to realize in usual fiber lasers because of cavity length and nonlinear effects. Hence, in comparison with fiber lasers, crystalline fiber lasers show great potential for high average power, pulse energy, and peak power.
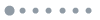
Figure 5.Typical pulse trains and single-pulse profile at (a), (b) threshold and (c), (d) maximum output power, respectively.
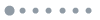
Figure 6.Dependences of (a) pulse width, (b) pulse repetition rate, (c) pulse energy, and (d) pulse peak power on pump powers.
Last but not least, we inserted a 0.1 mm BK7 thin plate acting as an F-P etalon into the laser resonator close to the saturable absorber. Slightly tilting the BK7 etalon, the pulsed laser can be wavelength tuned from 1833.20 to 1836.04 nm, i.e., a wavelength tuning range of about 2.84 nm, as plotted in Fig. 7. The relatively broad tuning range is impossible to achieve from other emission channels of Nd:YAG. On the one hand, it reflects that this specific transition band is broader than other bands for Nd:YAG. On the other hand, the present intense pumping-induced high gain favored this broad tuning, which is difficult to achieve from conventional Nd:YAG bulk lasers. The wavelength tuning indicates that a novel ultrashort pulsed eye-safe source at 1834 nm could be realizable by using the Nd:YAG single-crystal fiber via mode-locking technology.
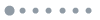
Figure 7.Wavelength tuning of -switched Nd:YAG lasers around 1834 nm.
4. CONCLUSION
To summarize, an 808 nm diode-end-pumped Nd:YAG single-crystal fiber laser at 1834 nm is demonstrated, and 3.28 W continuous-wave and 1.66 W Cr:ZnSe-based passively -switched laser operation are achieved. Slope efficiencies with respect to pump powers are 8.7% for the continuous-wave laser and 4.9% for the -switched laser. The shortest pulse width is about 104 ns, the corresponding maximum pulse energy is about 266.9 μJ, and the maximum pulse peak power is 2.54 kW. Note that the present results have not been optimized. Further optimizing transmission of the output coupler, the dopant concentration, and length of the Nd:YAG single crystal fiber, as well as the saturable absorber with appropriate initial transmission, better laser performance with pulse energy close to mJ scale could be expected.
References
[1] F. Gibert, D. Edouart, C. Cenac, F. Le Mounier. 2-μm high-power multiple-frequency single-mode Q-switched Ho:YLF laser for DIAL application. Appl. Phys. B, 116, 967-976(2014).
[2] W. Kim, S. R. Bowman, C. Baker, G. Villalobos, B. Shaw, B. Sadowski, M. Hunt, I. Aggarwal, J. Sanghera. Holmium doped laser materials for eye-safe solid state laser application. Proc. SPIE, 9081, 908105(2014).
[3] P. L. Luo, C. C. Kuo, C. C. Lee, J. T. Shy. Frequency stabilization of a single-frequency volume Bragg grating-based short-cavity Tm:Ho:YLF laser to a CO2 line at 2.06 μm. Appl. Phys. B, 109, 327-331(2012).
[4] R. C. Stoneman, L. Esterowitz. Efficient 1.94-μm Tm:YAlO laser. IEEE. J. Sel. Top. Quantum Electron., 1, 78-81(1995).
[5] R. Moncorgé, B. Chambon, J. Y. Rivoire, N. Garnier, E. Descroix, P. Laporte, H. Guillet, S. Roy, J. Mareschal. Nd-doped crystals for medical laser applications. Opt. Mater., 8, 109-119(1997).
[6] S. A. Payne, L. L. Chase, L. K. Smith, W. L. Kway. Infrared cross section measurements for crystals doped with Er3+, Tm3+, and Ho3+. IEEE J. Quantum Electron., 28, 2619-2630(1992).
[7] H. Zhou, X. Ma, Y. Wang, Z. Y. You, C. Y. Tu. Tm3+-doped Gd3Ga5O12 crystal: a potential tunable laser crystal at 2.0 μm. J. Alloys Compd., 475, 555-559(2009).
[8] X. D. Xu, X. D. Wang, Z. F. Lin, Y. Cheng, D. Z. Li, S. S. Cheng, F. Wu, Z. W. Zhao, C. Q. Gao, M. W. Gao, J. Xu. Crystal growth, spectroscopic and laser properties of Tm:LuAG crystal. Laser Phys., 19, 2140-2143(2009).
[9] R. W. Wallace. Oscillation of the 1.833-μ line in Nd3+:YAG. IEEE J. Quantum Electron., 7, 203-204(1971).
[10] T. S. Kubo, T. J. Kane. Diode-pumped lasers at five eye-safe wavelengths. IEEE J. Quantum Electron., 28, 1033-1040(1992).
[11] J. I. Mackenzie, C. Li, D. P. Shepherd. Multi-watt, high efficiency, diffraction-limited Nd:YAG planar waveguide laser. IEEE J. Quantum Electron., 39, 493-500(2003).
[12] J. I. Mackenzie, J. W. Szela, S. J. Beecher, T. L. Parsonage, R. W. Eason, D. P. Shepherd. Crystal planar waveguides, a power scaling architecture for low-gain transitions. IEEE J. Sel. Top. Quantum Electron., 21, 380-389(2015).
[13] B. Xu, H. Y. Xu, Z. P. Cai, R. Moncorgé. Watt-level narrow-linewidth Nd:YAG laser operating on 4F3/2→4I15/2 transition at 1834 nm. Opt. Express, 24, 3601-3606(2016).
[14] M. Frede, R. Wilhelm, D. Kracht. 250 W end-pumped Nd:YAG laser with direct pumping into the upper laser level. Opt. Lett., 31, 3618-3619(2006).
[15] X. Délen, I. Martial, J. Didierjean, D. Sangla, F. Balembois, P. Georges. 34 W continuous wave Nd:YAG single crystal fiber laser emitting at 946 nm. Appl. Phys. B, 104, 1-4(2011).
[16] D. Sangla, F. Balembois, P. Georges. Nd:YAG laser diode-pumped directly into the emitting level at 938 nm. Opt. Express, 17, 10091-10097(2009).
[17] K. Lebbou, A. Brenier, O. Tillement, J. Didierjean, F. Balembois, P. Georges, D. Perrodin, J. M. Fourmigue. Long (111)-oriented Y3Al5O12:Nd3+ single crystal fibers grown by modified micro-pulling down technology for optical characterization and laser generation. Opt. Mater., 30, 82-84(2007).
[18] J. Didierjean, F. Balembois, P. Georges, D. Perrodin, K. Lebbou, A. Brenier, O. Tillemen. High-power laser with Nd:YAG single-crystal fiber grown by the micro-pulling-down technique. Opt. Lett., 31, 3468-3470(2006).
[19] X. Délen, S. Piehler, J. Didierjean, N. Aubry, M. A. Ahmed, T. Graf, F. Balembois, P. Georges. 250 W single-crystal fiber Yb:YAG laser. Opt. Lett., 37, 2898-2900(2012).
[20] D. Sangla, I. Martial, N. Aubry, J. Didierjean, D. Perrodin, F. Balembois, K. Lebbou, A. Brenier, P. Georges, O. Tillement, J. Fourmigué. High power laser operation with crystal fibers. Appl. Phys. B, 97, 263-273(2009).
[21] A. Aubourg, J. Didierjean, N. Aubry, F. Balembois, P. Georges. Passively Q-switched diode-pumped Er:YAG solid-state laser. Opt. Lett., 38, 938-940(2013).
[22] H. Kim, R. S. Hay, S. A. McDaniel, G. Cook, N. G. Usechak, A. M. Urbas, K. N. Shugart, H. Lee, A. H. Kadhim, D. P. Brown, B. Griffin, G. E. Fair, R. G. Corns, S. A. Potticary, F. K. Hopkins, K. L. Averett, D. E. Zelmon, T. A. Parthasarathy, K. A. Keller. Lasing of surface-polished polycrystalline Ho:YAG (yttrium aluminum garnet) fiber. Opt. Express, 25, 6725-6731(2017).
[23] P. Koranda, J. Šulc, M. Doroshenko, H. Jelínková, T. T. Basiev, V. Osiko, V. V. Badikov. Cr:ZnSe laser pumped with Tm:YAP microchip laser. Proc. SPIE, 7578, 757826(2010).
[24] J. L. Lan, X. F. Guan, B. Xu, R. Moncorgé, H. Y. Xu, Z. P. Cai. A diode-pumped Tm:CaYAlO4 laser at 1851 nm. Laser Phys. Lett., 14, 075801(2017).
[25] L. D. DeLoach, R. H. Page, G. D. Wilke, S. A. Payne, W. F. Krupke. Transition metal-doped zinc chalcogenides: spectroscopy and laser demonstration of a new class of gain media. IEEE J. Quantum Electron., 32, 885-895(1996).
[26] H. C. Lee, S. U. Byeon, A. Lukashev. Diode-pumped continuous-wave eye-safe Nd:YAG laser at 1415 nm. Opt. Lett., 37, 1160-1162(2012).
[27] J. L. Lan, Z. Y. Zhou, X. F. Guan, B. Xu, H. Y. Xu, Z. P. Cai. New continuous-wave and Q-switched eye-safe Nd:YAG lasers at 1.4 μm spectral region. J. Opt., 19, 045504(2017).