
- Chinese Optics Letters
- Vol. 20, Issue 10, 103602 (2022)
Abstract
1. Introduction
A light beam carrying orbital angular momentum (OAM) with a helical wavefront, which can be represented as
2. Results and Discussion
The distribution of our BMVG can be simply expressed as
Figure 1.Concept and working principle of our rGO broadband multifocal vortex generator (BMVG). (a) Schematic of working principle of our BMVG. (b) Simulated intensity and phase profiles of continuous OAM patterns captured at corresponding focal planes. Scale bar, 4 µm.
Here,
Sign up for Chinese Optics Letters TOC. Get the latest issue of Chinese Optics Letters delivered right to you!Sign up now
As is shown in Fig. 1(a), the zone plate structure defined by Eq. (3) can generate multiple foci with multiple deep depths of foci (DoFs) at focal planes of
To illustrate our idea, we design our BMVG according to Eq. (1) by choosing
To demonstrate that the generated OAMs from our BMVG have deep DoFs, we simulated the intensity profile of the light field in the
Figure 2.Simulated light fields of our BMVG in the x–z plane. (a) Simulated intensity profile in the x–z plane of the light field generated by our BMVG. (b) Zoom-in simulated intensity profiles (x–z plane) of the non-diffracting regions of generated OAM modes from l
To further verify the function of our device, we experimentally fabricated and characterized the designed BMVG. We fabricated our designed BMVG on a 1-µm-thick reduced graphene oxide (rGO) film[
Figure 3.Fabricated sample and measured results of the BMVG. (a) The scanning electronic microscopy (SEM) image of the entire fabricated sample. (b) The topographic profile (top) of the rGO sample measured by an optical profiler and the cross-sectional profile (bottom) marked by the white dashed line in the top image of (b). (c) The interference patterns of the generated OAMs captured before and after the designed focal planes upon 633 nm illumination. Scale bar, 6 µm.
Figure 3(c) shows a series of interference patterns captured before and after the centers of the non-diffracting regions of different modes. According to the interference patterns, we can directly read the modes of the generated OAMs from the number of arms in the intensity profiles. For each mode, the handedness of the interference patterns before and after the center of the non-diffracting region is opposite. This is because before the beginning of the non-diffracting region of each mode the wavefront of the light wave coming from our designed BMVG is convex [as is shown in Fig. 2(a)]. After the non-diffracting region, it will then diverge, and the wavefront will become concave. Such a phase change in the beam leads to the change of handedness of the interference patterns. But, the handedness of the OAMs never changes during this process. From Fig. 3(c), the interference patterns from
The unique properties of our BMVG also facilitate it to work as a wavelength tunable and focal distance tunable device. Specifically, Eq. (1) can be rewritten in a different formation as
Figure 4.Demonstration of wavelength tunability and focal distance tunability of our BMVG. (a) Simulated and measured interference patterns of generated OAMs captured before (left panel) and after (right panel) z = 450 µm under the incident light of 886 nm, 739 nm, and 633 nm. Scale bar, 6 µm. (b) The measured interference patterns of the generated OAMs under 633 nm (near z = 720 µm), 760 nm (near z = 600 µm), 800 nm (near z = 570 µm), 850 nm (near z = 536 µm), 886 nm (near z = 514 µm), 900 nm (near z = 506 µm), and 1000 nm (near z = 456 µm). Scale bar, 6 µm. (c) The measured interference patterns of the generated OAMs captured before and after the designed focal planes upon 900 nm illumination. Scale bar, 6 µm.
First of all, we tested the wavelength tunability of the device. We fixed the test position before the focal plane at
3. Conclusion
In conclusion, we proposed a multi-turn spiral slit structure, which naturally embodies the structure of a continuous spiral slit vortex generator and a zone plate. Such a device can work as a broadband multifocal continuous vortex generator. We prove the function of our device with rigorous mathematical language and completely consistent simulation and experimental results. Compared with previous similar devices, our BMVG can work with both linearly polarized light and circularly polarized light at different wavelengths. In addition, it can be fabricated by a simple and scalable laser nanofabrication method and takes much less fabrication time. The flexibility of our design enables it to work under a broadband spectrum while working as a wavelength tunable and focal position tunable device. However, our device’s efficiency is low since a majority of the incident light is blocked by the rGO film. Nevertheless, overall, we believe the multi-functionalities and tuning flexibility of our graphene BMVG provide a new avenue for next generation scalable OAM generators.
4. Methods
Simulation: The simulation of this work is based on the Fresnel diffraction theory and the codes are implemented on MATLAB on a personal computer.
Fabrication: A 1-µm-thick graphene oxide (GO) film fabricated by the vacuum filtration method[
The vortex graphene generator is fabricated in one step by a commercial laser nanoprinting setup (Special Edition, Nanoprint3D from Innofocus Photonics Technology Pty. Ltd.) with a femtosecond laser (Libra, 800 nm wavelength, 100 fs pulse, 10 kHz repetition rate). The computer-controlled system was used to control the parameters of the laser ablation process, including exposure time, laser power, scanning speed, and patterns. The scanning speed was 100 µm/s to ensure a smooth line fabrication. The laser focal spot has a full-width at half-maximum (FWHM) of 600 nm for presenting high resolution. The laser power is 100 µW.
Optical characterization: The focusing performance of the graphene generator is characterized by a home-made imaging system, which uses a
References
[1] H. Sroor, Y.-W. Huang, B. Sephton, D. Naidoo, A. Vallés, V. Ginis, C.-W. Qiu, A. Ambrosio, F. Capasso, A. Forbes. High-purity orbital angular momentum states from a visible metasurface laser. Nat. Photonics, 14, 498(2020).
[2] C. Huang, C. Zhang, S. Xiao, Y. Wang, Y. Fan, Y. Liu, N. Zhang, G. Qu, H. Ji, J. Han, L. Ge, Y. Kivshar, Q. Song. Ultrafast control of vortex microlasers. Science, 367, 1018(2020).
[3] Z. Jin, D. Janoschka, J. Deng, L. Ge, P. Dreher, B. Frank, G. Hu, J. Ni, Y. Yang, J. Li, C. Yu, D. Lei, G. Li, S. Xiao, S. Mei, H. Giessen, F. Meyer zu Heringdorf, C.-W. Qiu. Phyllotaxis-inspired nanosieves with multiplexed orbital angular momentum. eLight, 1, 5(2021).
[4] S. Mei, K. Huang, T. Zhang, M. Qasim Mehmood, H. Liu, C. T. Lim, J. Teng, C.-W. Qiu. Evanescent vortex: optical subwavelength spanner. Appl. Phys. Lett., 109, 191107(2016).
[5] Y. Shen, X. Wang, Z. Xie, C. Min, X. Fu, Q. Liu, M. Gong, X. Yuan. Optical vortices 30 years on: OAM manipulation from topological charge to multiple singularities. Light Sci. Appl., 8, 90(2019).
[6] G. Chen, Z.-Q. Wen, C.-W. Qiu. Superoscillation: from physics to optical applications. Light Sci. Appl., 8, 56(2019).
[7] R. Zeng, Q. Zhao, Y. Shen, Y. Liu, Y. Yang. Structural stability of open vortex beams. Appl. Phys. Lett., 119, 171105(2021).
[8] Q. Zhou, M. Liu, W. Zhu, L. Chen, Y. Ren, H. J. Lezec, Y. Lu, A. Agrawal, T. Xu. Generation of perfect vortex beams by dielectric geometric metasurface for visible light. Laser Photonics Rev., 15, 2100390(2021).
[9] N. Zhou, S. Zheng, X. Cao, Y. Zhao, S. Gao, Y. Zhu, M. He, X. Cai, J. Wang. Ultra-compact broadband polarization diversity orbital angular momentum generator with 3.6 × 3.6 µm2 footprint. Sci. Adv., 5, eaau9593(2019).
[10] L. Jin, Y.-W. Huang, Z. Jin, R. C. Devlin, Z. Dong, S. Mei, M. Jiang, W. T. Chen, Z. Wei, H. Liu, J. Teng, A. Danner, X. Li, S. Xiao, S. Zhang, C. Yu, J. K. W. Yang, F. Capasso, C.-W. Qiu. Dielectric multi-momentum meta-transformer in the visible. Nat. Commun., 10, 4789(2019).
[11] Y. Yang, L. Wu, Y. Liu, D. Xie, Z. Jin, J. Li, G. Hu, C.-W. Qiu. Deuterogenic plasmonic vortices. Nano Lett., 20, 6774(2020).
[12] S. Mei, M. Q. Mehmood, S. Hussain, K. Huang, X. Ling, S. Y. Siew, H. Liu, J. Teng, A. Danner, C.-W. Qiu. Flat helical nanosieves. Adv. Funct. Mater., 26, 5255(2016).
[13] H. Lin, B. Jia, M. Gu. Dynamic generation of Debye diffraction-limited multifocal arrays for direct laser printing nanofabrication. Opt. Lett., 36, 406(2011).
[14] Y. Yang, H. Lin, B. Y. Zhang, Y. Zhang, X. Zheng, A. Yu, M. Hong, B. Jia. Graphene-based multilayered metamaterials with phototunable architecture for on-chip photonic devices. ACS Photonics, 6, 1033(2019).
[15] G. Cao, H. Lin, S. Fraser, X. Zheng, B. Del Rosal, Z. Gan, S. Wei, X. Gan, B. Jia. Resilient graphene ultrathin flat lens in aerospace, chemical, and biological harsh environments. ACS Appl. Mater. Interfaces, 11, 20298(2019).
[16] H. Lin, B. C. P. Sturmberg, K.-T. Lin, Y. Yang, X. Zheng, T. K. Chong, C. M. de Sterke, B. Jia. 90-nm-thick graphene metamaterial for strong and extremely broadband absorption of unpolarized light. Nat. Photonics, 13, 270(2019).
[17] X. Zheng, B. Xu, S. Li, H. Lin, L. Qiu, D. Li, B. Jia. Free-standing graphene oxide mid-infrared polarizers. Nanoscale, 12, 11480(2020).
[18] X. Zheng, B. Jia, H. Lin, L. Qiu, D. Li, M. Gu. Highly efficient and ultra-broadband graphene oxide ultrathin lenses with three-dimensional subwavelength focusing. Nat. Commun., 6, 8433(2015).
[19] H. Wang, C. Hao, H. Lin, Y. Wang, T. Lan, C.-W. Qiu, B. Jia. Generation of super-resolved optical needle and multifocal array using graphene oxide metalenses. Opto-Electron. Adv., 4, 02200031(2021).
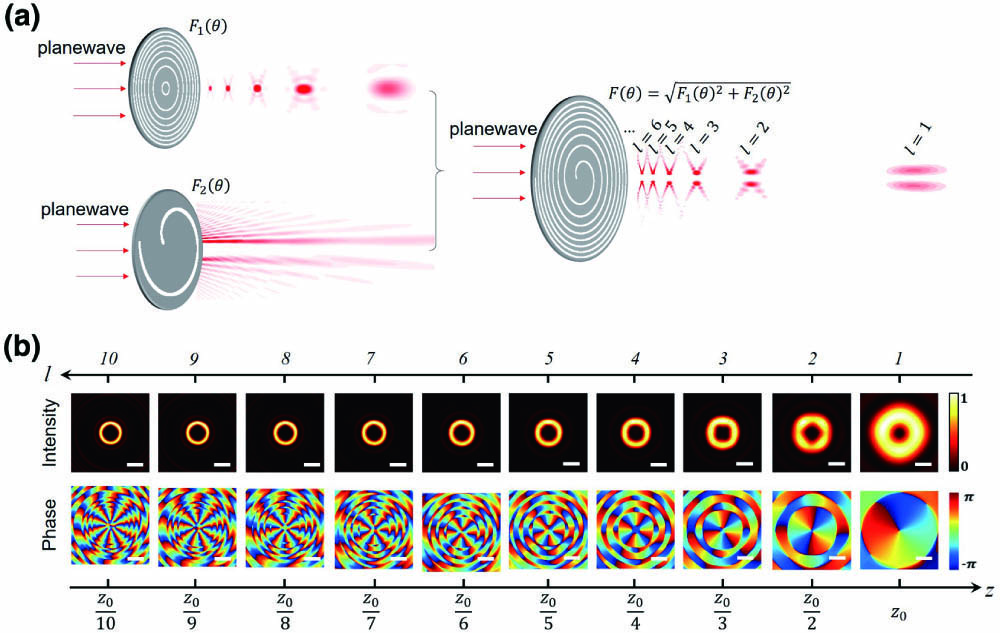
Set citation alerts for the article
Please enter your email address