High magnetic field spectroscopy has been performed on lead chloride-based perovskite, a material that attracts significant interest for photovoltaic and photonic applications within the past decades. Optical properties being mainly driven by the exciton states, we have measured the fundamental parameters, such as the exciton binding energy, effective mass, and dielectric constant. Among the inorganic halide perovskites,

- Photonics Research
- Vol. 8, Issue 10, A50 (2020)
Abstract
1. INTRODUCTION
Halide perovskite semiconductors have emerged as promising materials for photovoltaic and optoelectronic applications. Within the last decade, the conversion efficiency of perovskite solar cells has jumped in an impressive manner from 3% to 25% [1–3]. These outstanding results have also fueled interest on these materials in other domains. As a direct band gap semiconductor, lead halide perovskite also exhibits excellent electronic and emission properties, very interesting for optoelectronic applications as lasers and photodiodes [4–7], photodetectors [8], or polaritonic devices [9–13]. In addition, the flexible synthesis of nanostructures, such as nanocrystals or nanoplatelets, offers numerous opportunities for their integration in quantum devices and the development of applications in the field of quantum optics [14–16].
Emission properties being driven by the excitonic state, a direct experimental determination of the exciton parameters such as binding energy, effective mass, and Landé factor, is of prime importance for current understanding and future technological applications. Indeed, the theoretical models describing the exciton fine structure or describing the exciton magnetic behavior are based on the direct knowledge of the exciton parameters [17–22]. From the experimental point of view, getting accurate values of the binding energy implies that we can perform and analyze spectroscopic studies on these materials based on techniques such as absorption, photoluminescence, or reflectivity. Once the excitonic parameters are deduced, they allow for the interpretation of results obtained in more complex optical experiments such as time-resolved or photo-induced Faraday rotation measurements [23,24]. From the point of view of applications, the binding energy has to be compared with the thermal energy at the device operating point; it will determine whether the exciton will be stable or dissociate into electron and hole free carriers. The exciton stability is also important for polaritonic devices and room temperature (RT) single photon sources or lasers, while, for photovoltaic devices, the exciton dissociation is more interesting.
Here, we center our study on
Sign up for Photonics Research TOC. Get the latest issue of Photonics Research delivered right to you!Sign up now
In this study, we implement low-temperature transmission spectroscopy in pulsed magnetic fields up to 68 T, giving direct access to the basic exciton parameters, such as binding energy of the neutral free exciton and the excitonic reduced effective mass,
2. EXPERIMENTAL RESULTS
A typical optical microscopy image of grown films is shown in Fig. 1(a). The film thickness is in the 200–300 nm range. We observe strongly elongated parallelepipedal shaped domains with lateral dimensions of several tens of micrometers (
Figure 1.(a) Typical optical microscopy (reflection configuration) image of a
Figure 1(b) shows the optical transmission of
Compound | |||||
3.056 | 0.8 | ||||
2.342 | 7.3 | ||||
1.723 | 10.0 | ||||
2.233 | 22 | 0.115 | 8.42 | ||
1.501 | 14 | 0.09 | 9.35 | 2.3 | |
2.292 | 25 | 0.117 | 7.5 | ||
1.652 | 16 | 0.104 | 9.4 |
Table 1. Exciton Parameters in the Family of Halide Perovskite Compounds Deduced from Magneto-Optical Experiments at 2 K
Transmission measurements obtained at 2 K in high-magnetic fields are shown in Fig. 2(a). These measurements were performed in a Faraday configuration with pulsed magnetic fields up to 68 T (pulse duration 500 ms) in Toulouse at the Laboratoire National des Champs Magnétiques Intenses (LNCMI). An optical fiber was used to deliver white light from a xenon lamp. The focusing spot radius was 100–200 μm, allowing for the excitation of several domains. The circular polarization was introduced in situ. Rotation between
Figure 2.(a) Optical transmittance of the
In a magnetic field, the exciton energy is modified and can be written as
Fitting simultaneously the energy position of the
When the cyclotron energy is significantly smaller than the exciton binding energy,
We then obtain
The comparison of the exciton reduced mass, exciton binding energy, and effective dielectric screening for perovskites with different halides is presented in Fig. 3. The exciton binding energy increases with increasing band gap as in more conventional semiconductors (see also Fig. 4). At the same time, it can be seen that the effective exciton mass follows reasonably the prediction of a simple two-band
Figure 3.(a) Exciton reduced mass, (b) exciton binding energy, and (c) effective dielectric constant as functions of the energy gap. Measurements are done at 2 K. Full orange stars correspond to
Figure 4.(a) Exciton binding energy
The dielectric screening is shown in Fig. 3(c). It follows the expected trend, i.e., at low temperature when the motion of cations is frozen, the metal halide cage is determined in the first approximation of the screening properties. The dielectric constant then decreases when the bromide or iodide is exchanged with lighter Cl atoms [37,41]. Such a substitution increases the energy of phonon modes [43], since this energy is proportional to the inverse of the reduced mass of atoms participating in the vibration. As we can see in Fig. 3(c), the substitution of Pb atoms by Sn atoms results also in a decrease of the dielectric screening. Then, Fig. 3(c) shows that the effective dielectric constant decreases as the reduced mass of atoms building the crystal decreases and, consequently, a non-monotonous behavior of the dielectric constant versus the energy gap of the considered perovskite compounds.
Putting together arguments given above and the latter discussion, we underline that the substitution of
To finish, we have compared the exciton binding energy
3. CONCLUSION
We have performed a detailed magneto-optical study of the all-inorganic halide perovskite
References
[1] H. Zhou, Q. Chen, G. Li, S. Luo, T.-B. Song, H.-S. Duan, Z. Hong, J. You, Y. Liu, Y. Yang. Interface engineering of highly efficient perovskite solar cells. Science, 345, 542-546(2014).
[2] W. S. Yang, J. H. Noh, N. J. Jeon, Y. C. Kim, S. Ryu, J. Seo, S. Il Seok. High-performance photovoltaic perovskite layers fabricated through intermolecular exchange. Science, 348, 1234-1237(2015).
[3] . Best-research-cell efficiencies(2020).
[4] S. D. Stranks, H. J. Snaith. Metal-halide perovskites for photovoltaic and light-emitting diodes. Nat. Nanotechnol., 10, 391-402(2015).
[5] B. R. Sutherland, E. H. Sargent. Perovskite photonic sources. Nat. Photonics, 10, 295-302(2016).
[6] Q. Zhang, S. T. Ha, X. Liu, T. C. Sum, Q. Xiong. Room-temperature near-infrared high
[7] K. Lin, J. Xing, L. N. Quan, F. Pelayo Garcia de Arquer, X. Gong, J. Lu, L. Xie, W. Zhao, D. Zhang, C. Yan, W. Li, X. Liu, Y. Lu, J. Kirman, E. H. Sargent, Q. Xiong, Z. Wei. Perovskite light-emitting diodes with external quantum efficiency exceeding 20 percent. Nature, 562, 245-248(2018).
[8] L. T. Dou, Y. (Micheal) Yang, J. B. You, Z. R. Hong, W. H. Chang, G. Li, Y. Yang. Solution-processed hybrid perovskite photodetectors with high detectivity. Nat. Commun., 5, 5404(2014).
[9] R. Su, C. Diederichs, J. Wang, T. C. H. Liew, J. Zhao, S. Liu, W. Xu, Z. Chen, Q. Xiong. Room-temperature polariton lasing in all-inorganic perovskites nanoplatelets. Nano Lett., 17, 3982-3988(2017).
[10] P. Bouteyre, H. S. Nguyen, J. S. Lauret, G. Trippe-Allard, G. Delport, F. Ledee, H. Diab, A. Belarouci, C. Seassal, D. Garrot, F. Bretenaker, E. Deleporte. Room-temperature cavity polaritons with 3D hybrid perovskite: toward large-surface polaritonic devices. ACS Photon., 6, 1804-1811(2019).
[11] S. Zhang, J. Chen, J. Shi, L. Fu, W. Du, X. Sui, Y. Mi, Z. Jia, F. Liu, J. Shi, X. Wu, N. Tang, Q. Zhang, X. Liu. Trapped exciton-polariton condensate by spatial confinement in a perovskite microcavity. ACS Photon., 7, 327-337(2020).
[12] R. Su, J. Wang, J. Zhao, J. Xing, W. Zhao, C. Diederichs, T. C. H. Liew, Q. Xiong. Room temperature long-range coherent exciton polariton condensate flow in lead halide perovskites. Sci. Adv., 4, eaau0244(2018).
[13] R. Sui, S. Ghosh, J. Wang, S. Liu, C. Diederichs, T. C. H. Liew, Q. Xiong. Observation of exciton polariton condensation in perovskite lattice at room temperature. Nat. Phys., 16, 301-306(2020).
[14] Y.-S. Park, S. Guo, N. S. Makarov, V. I. Klimov. Room temperature single-photon emission from individual perovskite quantum dots. ACS Nano, 9, 10386-10393(2015).
[15] F. Hu, H. Zhang, C. Sun, C. Yin, B. Lv, C. Zhang, W. W. Yu, X. Wang, Y. Zhang, M. Xiao. Superior optical properties of perovskite nanocrystals as single photon emitters. ACS Nano, 9, 12410-12416(2015).
[16] C. Huo, C. F. Fong, M. R. Amara, Y. Huang, B. Chen, H. Zhang, L. Guo, H. Li, W. Huang, C. Diederichs, Q. Xiong. Optical spectroscopy of single colloidal CsPbBr3 perovskite nanoplatelets. Nano Lett., 20, 3673-3680(2020).
[17] Z. G. Yu. Effective-mass model and magneto-optical properties in hybrid perovskites. Sci. Rep., 6, 28576(2016).
[18] J. Ramade, L. M. Andriambariarijaona, V. Steinmetz, N. Goubet, L. Legrand, T. Barisien, F. Bernardot, C. Testelin, E. Lhuillier, A. Bramati, M. Chamarro. Fine structure of excitons and electron-hole exchange energy in polymorphic CsPbBr3 single nanocrystals. Nanoscale, 10, 6393-6401(2018).
[19] P. C. Sercel, J. L. Lyons, D. Wickramaratne, R. Vaxenburg, N. Bernstein, A. L. Efros. Exciton fine structure in perovskite nanocrystals. Nano Lett., 19, 4068-4077(2019).
[20] R. Ben Aich, I. Saïdi, S. Ben Radhia, K. Boujdaria, T. Barisien, L. Legrand, F. Bernardot, M. Chamarro, C. Testelin. Bright-exciton splittings in inorganic cesium lead halide perovskite nanocrystals. Phys. Rev. Appl., 11, 034042(2019).
[21] R. Ben Aich, S. Ben Radhia, K. Boujdaria, M. Chamarro, C. Testelin. Multiband k·p model for tetragonal crystals: application to hybrid halide perovskite nanocrystals. J. Phys. Chem. Lett., 11, 808-817(2020).
[22] M. Baranowski, K. Galkowski, A. Surrente, J. Urban, L. Klopotowski, S. Mackowsk, D. K. Maude, R. Ben Aich, K. Boujdaria, M. Chamarro, C. Testelin, P. K. Nayak, M. Dollmann, H. J. Snaith, R. J. Nicholas, P. Plochocka. Giant fine structure splitting of the bright exciton in a bulk MAPbBr3 single crystal. Nano Lett., 19, 7054-7061(2019).
[23] L. Q. Phuong, Y. Yamada, M. Nagai, N. Maruyama, A. Wakamiya, Y. Kanemitsu. Free carriers versus excitons in CH3NH3PbI3 perovskite thin films at low temperatures: charge transfer from the orthorhombic phase to the tetragonal phase. J. Phys. Chem. Lett., 7, 2316-2321(2016).
[24] P. Odenthal, W. Talmadge, N. Gundlach, R. Wang, C. Zhang, D. Sun, Z. G. Yu, Z. V. Vardeny, Y. S. Li. Spin-polarized exciton quantum beating in hybrid organic-inorganic perovskites. Nat. Phys., 13, 894-900(2017).
[25] Q. Zhang, R. Su, X. Liu, J. Xing, T. C. Sum, Q. Xiong. High-quality whispering-gallery-mode lasing from cesium lead halide perovskite nanoplatelets. Adv. Funct. Mater., 26, 6238-6245(2016).
[26] X. X. He, P. Liu, H. H. Zhang, Q. Liao, J. N. Yao, H. B. Fu. Patterning multicolored microdisk laser arrays of cesium lead halide perovskite. Adv. Mater., 29, 1604510(2017).
[27] M. H. Huang, S. Mao, H. Feick, H. Yan, Y. Wu, H. Kind, E. Weber, R. Russo, P. Yang. Room-temperature ultraviolet nanowire nanolasers. Science, 292, 1897-1899(2001).
[28] W. Liu, Q. Lin, H. Li, K. Wu, I. Robel, J. M. Pietryga, V. I. Klimov. Mn2+-doped lead halide perovskite nanocrystals with dual-color emission controlled by halide content. J. Am. Chem. Soc., 138, 14954-14961(2016).
[29] D. Parobek, B. J. Roman, Y. Dong, H. Jin, E. Lee, M. Sheldon, D. H. Son. Exciton-to-dopant energy transfer in Mn-doped cesium lead halide perovskite nanocrystals. Nano Lett., 16, 7376-7380(2016).
[30] X. Yuan, S. H. Ji, M. C. De Siena, L. L. Fei, Z. Zhao, Y. J. Wang, H. B. Li, J. L. Zhao, D. R. Gamelin. Photoluminescence temperature dependence, dynamics, and quantum efficiencies in Mn2+-doped CsPbCI3 perovskite nanocrystals with varied dopant concentration. Chem. Mater., 29, 8003-8011(2017).
[31] K. Saiki, K. Ueno, T. Shimada, A. Koma. Application of van-der-Waals epitaxy to highly heterogeneous systems. J. Cryst. Growth, 95, 603-606(1989).
[32] M. I. Utama, Z. Peng, R. Chen, B. Peng, X. Xu, Y. Dong, L. M. Wong, S. Wang, H. Sun, Q. Xiong. Vertically aligned cadmium chalcogenide nanowire arrays on muscovite mica: a demonstration of epitaxial growth strategy. Nano Lett., 11, 3051-3057(2011).
[33] S. T. Ha, X. Liu, Q. Zhang, D. Giovanni, T. C. Sum, Q. Xiong. Synthesis of organic-inorganic lead halide perovskite nanoplatelets: towards high-performance perovskite solar cells and optoelectronic devices. Adv. Opt. Mater., 2, 838-844(2014).
[34] L. Protesescu, S. Yakunin, M. I. Bodnarchuk, F. Krieg, R. Caputo, C. H. Hendon, R. Xi Yang, A. Walsh, M. V. Kovalenko. Nanocrystals of cesium lead halide perovskites (CsPbX3, X
[35] H. Ito, J. Nakahara, R. Onaka. Magneto-optical study of the exciton states in CsPbCl3. J. Phys. Soc. Jpn., 47, 1927-1935(1979).
[36] D. Fröhlich, K. Heidrich, G. Trendel. Cesium-trihalogen-plumbates a new class of ionic semiconductors. J. Lumin., 18-19, 385-388(1979).
[37] Z. Yang, A. Surrente, K. Galkowski, A. Miyata, O. Portugall, R. J. Sutton, A. A. Haghighirad, H. J. Snaith, D. K. Maude, P. Plochocka, R. J. Nicholas. Impact of the halide cage on the electronic properties of fully inorganic cesium lead halide perovskites. ACS Energy Lett., 2, 1621-1627(2017).
[38] K. Galkowski, A. Mitioglu, A. Miyata, P. Plochocka, O. Portugall, G. E. Eperon, J. Tse-Wei Wang, T. Stergiopoulos, S. D. Stranks, H. J. Snaith, R. J. Nicholas. Determination of the exciton binding energy and effective masses for methylammonium and formamidinium lead tri-halide perovskite semiconductors. Energy Environ. Sci., 9, 962-970(2016).
[39] M. Hirasawa, T. Ishihara, T. Goto, K. Uchida, N. Miura. Magnetoabsorption of the lowest exciton in perovskite-type compound (CH3NH3)PbI3. Physica B, 201, 427-430(1994).
[40] P. C. Makado, N. C. McGill. Energy level of a neutral hydrogen-like system in a constant magnetic field of arbitrary strength. J. Phys. C, 19, 873-885(1986).
[41] K. Galkowski, A. Surrente, M. Baranowski, B. Zhao, Z. Yang, A. Sadhanala, S. Mackowski, S. D. Stranks, P. Plochocka. Excitonic properties of low-band-gap lead–tin halide perovskites. ACS Energy Lett., 4, 615-620(2019).
[42] M. A. Becker, R. Vaxenburg, G. Nedelcu, P. C. Sercel, A. Shabaev, M. J. Mehl, J. G. Michopoulos, S. G. Lambrakos, N. Bernstein, J. L. Lyons, T. Stöferle, R. F. Mahrt, M. V. Kovalenko, D. J. Norris, G. Rainò, A. L. Efros. Bright triplet excitons in caesium lead halide perovskites. Nature, 553, 189-193(2018).
[43] Y. Kang, S. Han. Intrinsic carrier mobility of cesium lead halide perovskites. Phys. Rev. Appl., 10, 044013(2018).
[44] S. T. A. G. Melissen, F. Labat, P. Sautet, T. Le Bahers. Electronic properties of PbX3CH3NH3 (X=Cl, Br, I) compounds for photovoltaic and photocatalytic applications. Phys. Chem. Chem. Phys., 17, 2199-2209(2015).
[45] M. Sendner, P. K. Nayak, D. A. Egger, S. Beck, C. Muller, B. Epding, W. Kowalsky, L. Kronik, H. J. Snaith, A. Pucci, R. Lovrincic. Optical phonons in methylammonium lead halide perovskites and implications for charge transport. Mater. Horiz., 3, 613-620(2016).
[46] B. Guzelturk, P. L. Hernandez Martinez, Q. Zhang, Q. Xiong, H. Sun, X. W. Sun, A. O. Govorov, H. V. Demir. Excitonics of semiconductor quantum dots and wires for lighting and displays. Laser Photon. Rev., 8, 73-93(2014).
[47] D. D. Sell, S. E. Stokowski, R. Dingle, J. V. DiLorenzo. Polariton reflectance and photoluminescence in high purity GaAs. Phys. Rev. B, 7, 4568-4586(1973).
[48] C. Neumaon, A. Notheand, N. O. Lipari. Two-photon magnetoabsorption of ZnTe, CdTE and GaAs. Phys. Rev. B, 37, 922-932(1988).
[49] T. K. Tran, W. Park, W. Tong, M. M. Kyi, B. K. Wagner, C. J. Summers. Photoluminescence properties of ZnS epilayers. Appl. Phys. Lett., 81, 2803-2809(1997).
[50] E. Silveira, J. A. Freitas, M. Kneissl, D. W. Treat, N. M. Johnson, G. A. Slack, L. J. Schowalter. Near-bandedge cathodoluminescence of an AlN homoepitaxial film. Appl. Phys. Lett., 84, 3501-3503(2004).
[51] H. Warlimont, W. Martienssen. Springer Handbook of Materials Data(2018).
[52] D. J. Chadi, M. L. Cohen. Correlation between the static dielectric constant and the minimum energy gap. Phys. Lett. A, 49, 381-382(1974).
[53] R. Dalven. Empirical relation between energy gap and lattice constant in cubic semiconductor. Phys. Rev. B, 8, 6033-6034(1973).
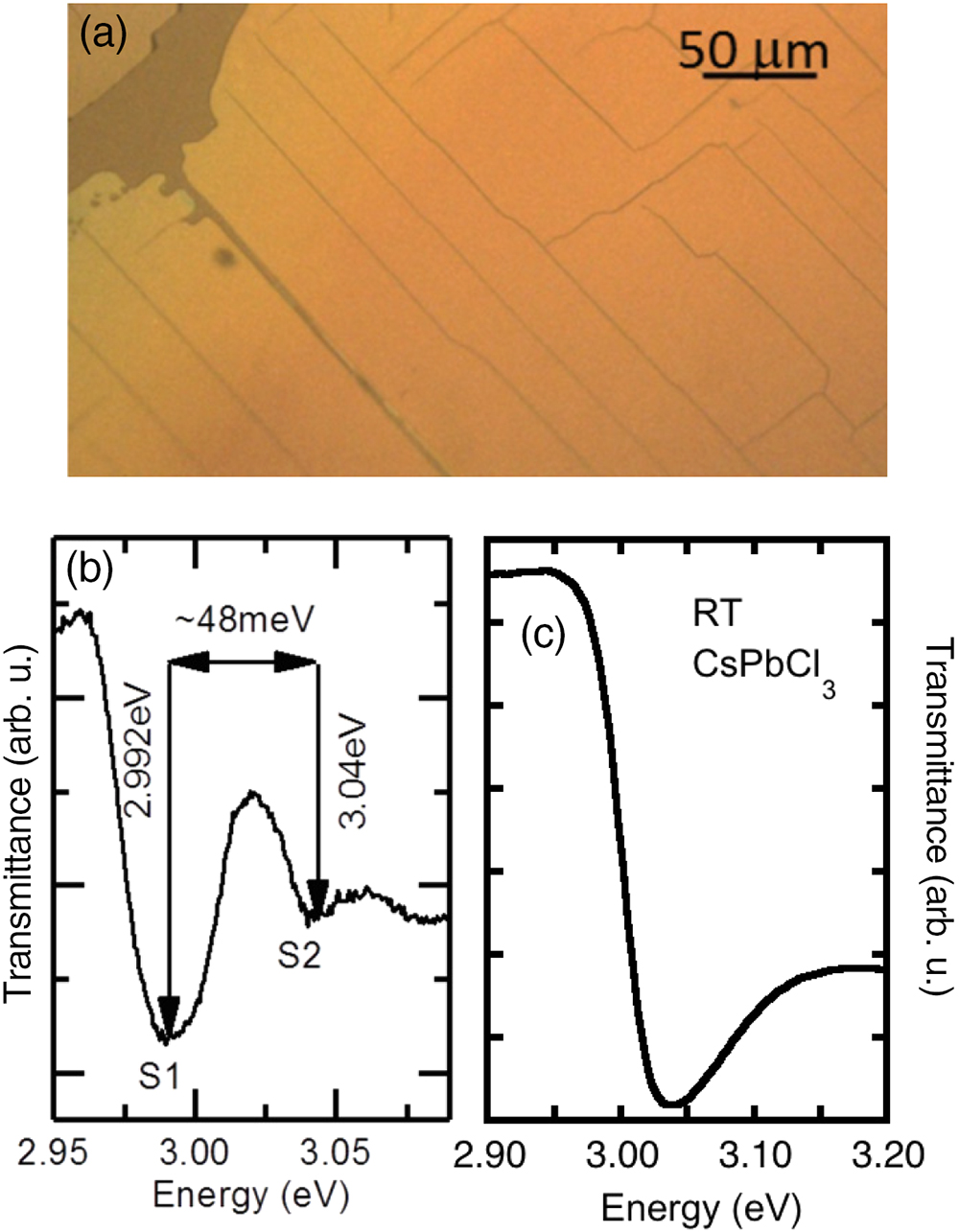
Set citation alerts for the article
Please enter your email address