
- Chinese Optics Letters
- Vol. 18, Issue 3, 031401 (2020)
Abstract
A single-frequency erbium-doped yttrium aluminum garnet (Er:YAG) laser operating at an eye-safe wavelength has drawn much attention in various applications like plastics processing, laser medicine, and wind measurement light detection and ranging (Lidar)[
For higher energy, a double gain-medium configuration has been used in recent years[
These double gain-medium high-energy lasers produce laser pulses with duration around 100 ns, while wind Lidar application requires laser pulses with a duration of hundreds of nanoseconds. Furthermore, for longer detection range, a higher-energy laser pulse is needed and will lead to shorter pulse duration, which will deteriorate the Lidar performance.
Sign up for Chinese Optics Letters TOC. Get the latest issue of Chinese Optics Letters delivered right to you!Sign up now
Besides, these single-frequency Er:YAG lasers are completely or partially pumped by a 1532 nm fiber laser, with an extra power transfer stage from a laser diode (LD) to an Er,Yb co-doped fiber laser, resulting in lower electrical to optical efficiency and a complicated system.
In this Letter, we demonstrate an LD pumped high-energy single-frequency Er:YAG laser at 1645 nm, with the pulse duration maintaining hundreds of nanoseconds. For high-energy laser pulses generation, we compared the common in-band pump source of a 1532 nm LD and 1470 nm LD theoretically. The analysis indicates that the 1470 nm LD outperforms the 1532 nm LD under high-energy operation. By using two 1470 nm LDs as pump sources and injection-locking technology, single-frequency laser pulses with pulse energy of 28.6 mJ, 21.6 mJ, and 15.0 mJ are obtained at PRFs of 200 Hz, 300 Hz, and 500 Hz. The pulse duration is maintained at 159 ns, 191 ns, and 263 ns, respectively, which satisfies the requirement of wind Lidar.
The center wavelengths of the in-band pump LD for the Er:YAG system are 1470 nm and 1532 nm, respectively. A simple model is used to compare the maximum upper-state density under different pumping wavelengths[
Item | Definition |
---|---|
Inversion density | |
Pump transition upper-state Boltzmann factor | |
Pump transition lower-state Boltzmann factor | |
Upper-state population density | |
Lower-state population density | |
Total dopant concentration | |
Normalized upper-state density |
Table 1. Definition of the Parameters
The inversion density of the pump transition is
In the pump transition process, the actual upper and lower states are
Define the normalized upper-state density as
Then, inversion density could be expressed as
For a laser system, the inversion density of the pump transition should be no greater than zero (the gain medium should absorb rather than emit the photons at the pump wavelength):
Figure 1.Maximum upper-state density versus different pump wavelengths.
As shown in Fig.
Besides, the absorption bandwidth of Er:YAG at room temperature is about 10 nm at 1470 nm, while it is much narrower (less than ∼1 nm) at 1532 nm[
Under the consideration above, we choose 1470 nm LDs as pump sources for our high-energy laser system.
The laser is an injection-locking system, consisting of a seed laser, a slave laser, and a control system. The laser system setup is shown in Fig.
Figure 2.Schematic diagram of laser setup. PD, photo detector; PBS, polarization beam splitter; HWP, half-wave plate; ISO, isolator; LD, laser diode; AOM, acousto-optic modulator; PZT, piezoelectric ceramic transducer.
The slave laser is a ring resonator with double gain media, composed by M1–M7. The gain media are two
For the ring cavity laser, the large reflection angle of the tilted spherical mirrors will result in astigmatism[
The Er:YAG ceramics in the slave laser are pumped by two fiber-coupled 1470 nm LDs, delivering 40 W output with 10 nm linewidth, respectively.
The diverging output from the fiber (
The seed laser is an LD pumped Er:YAG non-planar ring oscillator (NPRO), producing 150 mW stable continuous single-frequency output at 1645.22 nm, with a linewidth of less than 10 kHz. The seed laser beam is divided into two parts by a half-wave plate (HWP1) and a polarization beam splitter (PBS), for injection locking and heterodyne detection, respectively. The splitting ratio could be adjusted by HWP1. The s-polarized seed laser is rotated to be p-polarized and injected to the AOM at the first-order diffraction angle through M8. Lens 3 ensures the mode match between the injected master seed laser and the slave laser.
The injection locking is performed by the “Ramp-Fire” technique. Unfolded mirror M4 is mounted on a piezoelectric ceramic transducer (PZT), which is driven by the control system with a triangular wave. The leaked resonance signal is detected by the photo detector (PD1) behind M3. At the peak of the resonance signal, the cavity length meets the constructive interference condition of the seed laser frequency, and then the AOM is opened to build the laser pulses.
A small fraction of the output laser pulse is mixed with the seed laser for heterodyne detection, and the heterodyne signal is detected by PD2.
The
Figure 3.
After injection locking, the laser produces stable single-frequency pulses with slightly reduced pulse energy. The single-frequency laser output performance is shown in Fig.
Figure 4.Characteristics of single-frequency pulse energy and width versus pump power.
Figure
Figure 5.Build-up time with and without seed injection at a PRF of 200 Hz.
The heterodyne detection result of the maximum output energy at 200 Hz is shown in Fig.
Figure 6.Heterodyne detection result and FFT result at the highest output energy.
Figure 7.Central frequency stability of the beating signal.
As shown in Fig.
The stability of pulse energy under the output energy of 28.6 mJ is also tested in 30 min, with an RMS less than 2.1%.
The beam profile is measured in different positions by a Pyrocam III-C-A camera (Spricon Inc.) at the highest output energy (Fig.
Figure 8.Beam characterization at highest output energy.
In summary, an LD pumped, single-frequency high-energy Er:YAG laser system suitable for wind measurement Lidar is demonstrated. With the configuration of the double gain media pumped by 1470 nm LDs, up to 28.6 mJ single-frequency laser pulses with pulse duration of 159 ns at 1645 nm under a PRF of 200 Hz are obtained. The
References
[2] C. Q. Gao, L. N. Zhu, R. Wang, M. W. Gao, Y. Zheng, L. Wang. Opt. Lett., 37, 1859(2012).
[3] B. Q. Yao, X. Yu, X. L. Liu, X. M. Duan, Y. L. Ju, Y. Z. Wang. Opt. Express, 21, 8916(2013).
[5] R. C. Stoneman, R. Hartman, A. I. R. Malm, P. Gatt. Proc. SPIE, 5791, 167(2005).
[8] Y. Deng, X. Yu, B. Q. Yao, T. Y. Dai, X. M. Duan, Y. L. Ju. Laser Phys, 24, 045809(2014).
[14] Z. Z. Yu, M. J. Wang, X. Hou, W. B. Chen. Appl. Phys. B, 122, 84(2016).
[16] M. Zhang, C. Q. Gao, Q. Wang, M. W. Gao, S. Huang, K. X. Wang. Opt. Express, 27, 23197(2019).
[17] P. M. Burns, M. Chen, D. Pachowicz, S. Litvinovitch, F. Fitzpatrick, Ni. W. Sawruk. Applications of Lasers for Sensing and Free Space Communications, SW3H-2(2018).
[18] A. Sennaroglu. Solid-State Lasers and Applications(2007).
[19] I. Kudryashov, N. Ter-Gabrielyan, M. Dubinskii. Proc. SPIE, 7325, 732505(2009).
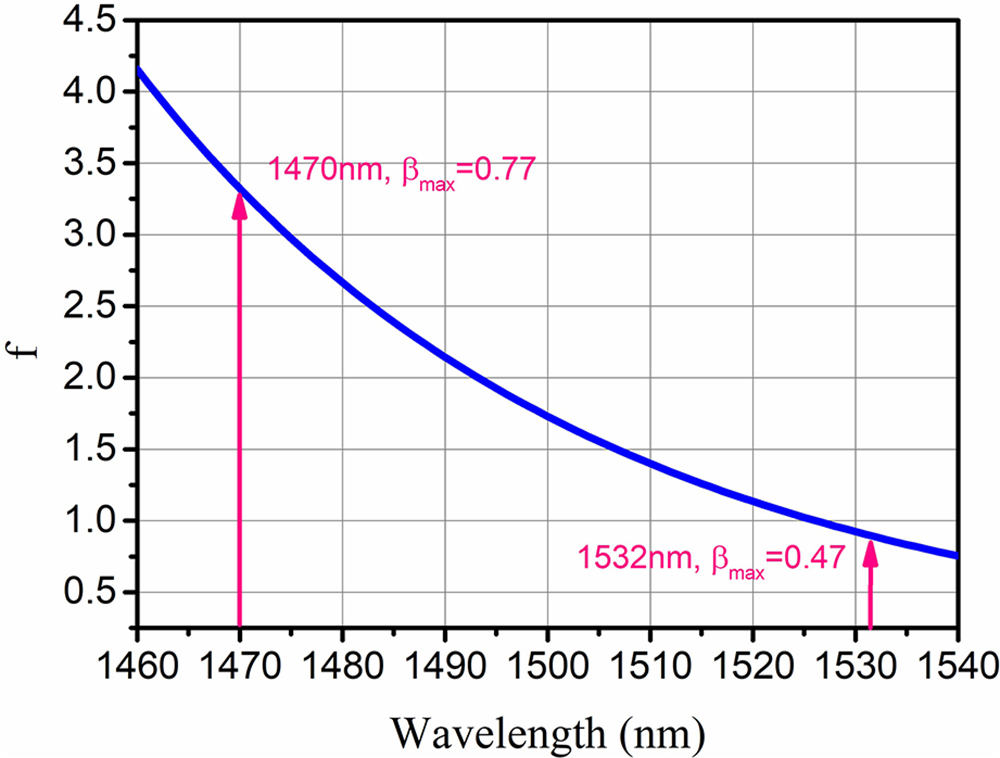
Set citation alerts for the article
Please enter your email address