Abstract
A high power continuous-wave single-frequency green fiber laser by second-harmonic generation of a Yb-doped fiber amplifier (YDFA) is developed. A linearly polarized single-mode fiber amplifier produces a 60 W infrared laser at 1064 nm with a 103 W incident diode pump laser at 976 nm, corresponding to an optical conversion efficiency of 58%. An external bow-tie enhancement cavity incorporating a noncritically phase-matched lithium triborate crystal is employed for second-harmonic generation. A 33.2 W laser at 532 nm is obtained with a 45 W incident 1064 nm fundamental laser, corresponding to a conversion efficiency of 74%.Continuous-wave (CW) green lasers have many scientific and industrial applications, including laser display[1,2], pumping of Ti-sapphire and dye lasers[3], semiconductor mask inspection[4], the generation of CW UV radiation by second-harmonic generation (SHG)[5], etc. Due to the lack of solid state gain medium that can directly lase at the green spectral range, the standard method for generating a green laser is nonlinear frequency conversion. Periodically poled lithium niobate/tantalite () or potassium titanyl phosphate (KTP) can be used to efficiently produce visible sources by a single-pass SHG of near-infrared lasers, which have a high effective nonlinear coefficient . But, they are prone to damage when the incident fundamental power is higher than multiple tens of watts[6–9]. For high power applications, the use of nonlinear crystals with a higher tolerance to optical power is necessary. Lithium triborate (LBO) is an excellent nonlinear crystal with a high damage threshold, which, however, has a smaller . Intracavity frequency doubling is commonly used in solid state lasers to improve the SHG efficiency by increasing the fundamental power incident on the nonlinear crystal. However, the thermal effects in the laser medium limit the output power and the optical beam quality[10,11].
Yb-doped fiber (YDF) lasers have advanced greatly in recent years, and are replacing bulk solid state lasers in many fields of application[12,13]. The fiber laser geometry is beneficial for thermal management, thus, it supports higher power operation[14,15]. So, the frequency doubling of a YDF laser is a superior route to produce high power green sources. Normal intracavity frequency doubling is not efficient for fiber lasers due to the limited intracavity power enhancement. A novel method of SHG in an enhancement resonator within a fiber laser cavity has been proposed and generated up to a 19 W output[16]. However, the laser stability and power scalability remain to be demonstrated. Instead, SHG in an external enhancement cavity is possibly a more scalable technique for generating a fiber-based visible laser, because it separates the fundamental laser and frequency doubling stage. With a 1064 nm single-frequency fiber master oscillator power amplifier (MOPA) and a resonant cavity for enhanced SHG in LBO, a more than 20 W CW single-frequency 532 nm laser was reported[17]. Similar results have been reported at other wavelengths[18]. The challenge for further power scaling is on increasing the power of the single-frequency YDF MOPA, which is usually limited by stimulated Brillouin scattering (SBS), and thermal management of the resonant doubling cavity.
In this Letter, we report a high power CW single-frequency 532 nm laser by frequency doubling of a YDF MOPA. The CW linearly polarized single-frequency YDF amplifier (YDFA) produces a 60 W laser at 1064 nm with an optical conversion efficiency of 58%. A bow-tie external resonant cavity incorporating an LBO nonlinear optical crystal is used for generating the second harmonic of the fiber laser. The LBO crystal is type-I noncritically phase matched, which avoids a walk-off effect between the fundamental and harmonic waves[19,20]. A 33.2 W green laser at 532 nm is generated with a 45 W incident fundamental laser. The corresponding conversion efficiency is 74%.
Sign up for Chinese Optics Letters TOC. Get the latest issue of Chinese Optics Letters delivered right to you!Sign up now
The experimental configuration of the green fiber laser is shown in Fig. 1. The seed source is a 1064 nm distributed feedback (DFB) diode laser (QD Laser, QLD1061) with a maximum fiber pigtailed output of 50 mW and a linewidth of 17.6 MHz. A modulating signal is loaded onto the power driver of the seed, which is used for frequency locking of the following enhancement cavity with the well-established Pound–Drever–Hall (PDH) method. The 1064 nm YDF pre-amplifier delivers up to a 360 mW CW laser. For the main amplifier, the pump sources are two 60 W diode lasers at 976 nm, which are coupled into the gain fiber with a combiner. The gain fiber for the main amplifier is 1.5 m of 10/125 μm polarization maintaining (PM) YDF, which has a core diameter of 10 μm, a numerical aperture (NA) of 0.085, and a cladding absorption of 5 dB/m at 976 nm. A PM isolator (ISO) is inserted between the seed and the pre-amplifier. A circulator is placed before the main amplifier to monitor the backscattered light, and plays the role of an ISO at the same time. The heat sink of the gain fiber is water-cooled. The output beam of the YDFA is collimated for the following SHG.
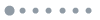
Figure 1.Schematic diagram of the frequency doubled CW single-frequency YDF MOPA.
The collimated 1064 nm fiber amplifier output is optically isolated, and then coupled into a homemade bow-tie ring resonant cavity with a pair of steering mirrors and a mode-matching lens. The resonant cavity consists of two plane mirrors (M1, M2) and two concave mirrors (M3, M4). The total geometrical cavity length is 157 mm. A 30-mm-long, noncritically phase-matched, antireflection-coated LBO crystal is installed in an oven and placed between two concave mirrors. Both mirrors have a curvature radius of 50 mm. In the experiment, the optimum crystal temperature is found to be 154.6°C, which is higher than the theoretical value of 149°C. This is because one side face of the crystal is exposed to ambient air of 20°C. The beam waist radius inside the LBO crystal is . The antireflection coating of the LBO has a reflectivity of . The input coupler has a transmission of 12% at 1064 nm, which is close to the optimum impedance-matching condition. The dichroic output coupler has a high transmittance at 532 nm (estimated: 98.06%) and a high reflection (HR) at 1064 nm (estimated: 99.89%).
In order to achieve efficient SHG, it also needs to ensure optimum overlap between the fundamental light beam and the eigenmode of the resonant cavity. The waist radius at the mid-point of the resonator arm defined by two plane mirrors is calculated to be . The focal length and the position of the quartz lens are carefully adjusted for efficient coupling. In addition, a half-wave plate is inserted between the fiber laser and the external cavity to match the polarization of the fiber output to the ordinary axis of the LBO crystal. The ring resonator is actively locked to the 1064 nm single-frequency laser using the PDH method.
Limited by the nonlinear effect of SBS, the power scaling has been difficult for a single-frequency fiber amplifier. So far, there are several methods for increasing the SBS threshold, for example, by using a large mode area (LMA) fiber[21], decreasing the gain fiber length[22], broadening the effective SBS gain spectrum[23], and so on. In the experiment, the SBS is avoided by using a Yb-doped gain fiber and a delivery fiber as short as possible. Only a 1.5 m gain fiber is used. The linearly polarized single-frequency 1064 nm laser output as a function of diode pump power is shown in Fig. 2. When the diode pump power reaches 103 W, the output power is 60 W, corresponding to an optical-optical conversion efficiency of . The efficiency is lower than usual 1064 nm YDFAs due to the lower pump absorption as a result of the short gain fiber. Meanwhile, the backward light starts to increase quickly to 34 mW.
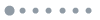
Figure 2.1064 nm output power and backward light power of the main amplifier as a function of the pump power.
The spectra of the collimated 1064 nm laser at different output powers are measured with an optical spectra analyzer (AQ6370), as shown in Fig. 3. The residual pump light is 40 dB lower than the signal light. There is a small amount of amplified spontaneous emission in the output, which is 60 dB lower than the laser, as seen in the zoom-in spectra in Fig. 3(b), measured with a resolution of 0.02 nm. The spectra in Fig. 3(b) are resolution limited. Figure 4 shows the backward light spectra at different output powers. It can be seen that the backward light consists of amplified spontaneous emission, Rayleigh scattering of the laser, and the SBS light. The Rayleigh scattering light and SBS light separate about 0.06 nm spectrally. At high power, the SBS light is much higher than the Rayleigh scattering light. But, the total power of the backward light is only 34 mW even at the highest output. The polarization extinction ratio (PER) of the amplifier output is measured to be , owing to the all PM fiber configuration.
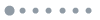
Figure 3.Output spectra of the 1064 nm fundamental laser at different output powers: (a) wide spectra, (b) fine spectra.
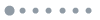
Figure 4.Spectra of the backward light from the 1064 nm main amplifier at different output powers: (a) wide spectra, (b) fine spectra.
The amplified single-frequency 1064 nm laser is frequency doubled in a resonant cavity described in the setup section. Mode-match lenses with various focal lengths are compared for efficient coupling. A lens with a focal length of 300 mm is finally chosen for high power experiments. The optimum position of the lens is fine-tuned experimentally. Figure 5 shows the dependence of the SHG output power and the conversion efficiency on the incident pump power. At low power, the 532 nm output increases nonlinearly as a result of the SHG process. At higher power, the frequency doubled output becomes linear with respect to the fundamental laser. Up to 33.2 W of 532 nm output is obtained when a 45 W 1064 nm laser is incident on the resonant cavity. The maximum fundamental laser incident on the cavity is lower than the 60 W direct amplifier output because of the cumulated optical losses in the ISO, steering mirrors, wave plates, collimator, and lens. The external conversion efficiency from 1064 nm to 532 nm is shown with the right axis in Fig. 5. The conversion efficiency at the highest power is 74%. By employing the theory of Ashkin, Boyd, and Dziedzic (ABD)[24], the dependence of the SHG output power on the pump power is simulated and fitted, as shown in Fig. 5. In the fitting, the single-pass conversion factor is calculated to be , and the total intracavity loss is set to 4%. However, the estimated total intracavity loss in the setup is about less than 1%, much lower than 4%. Therefore, it is believed that the SHG efficiency can be increased by further improving the mode matching between the incident beam and the cavity eigenmode. The mode matching is challenging at a high power due to the thermal effects in the optics as well as in the nonlinear crystals.
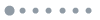
Figure 5.Dependence of the second-harmonic output power and the conversion efficiency on the pump power. A numerical fitting is also given.
The linewidth of the 1064 and 532 nm lasers are measured with a Fabry–Perot interferometer (FPI) of 4 GHz free spectral range for the respective wavelength region. As shown in Fig. 6, the 1064 nm laser has a linewidth of , while the linewidth of the 532 nm laser is . The measured linewidth is wider than usual reports for DFB diode lasers, which we believe is due to the homemade DFB diode laser driver. Since the fundamental laser is generated with a single-mode fiber, and the frequency doubling is achieved with an LBO crystal within a resonant cavity, near-diffraction-limited beam quality is expected for the 532 nm green laser. Figure 7 shows the far-field image of the 532 nm laser, which has a perfect Gaussian profile.
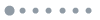
Figure 6.Linewidth measured with an FPI: (a) 1064 nm laser, (b) 532 nm laser.
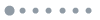
Figure 7.Far-field beam profile of the 532 nm laser.
In conclusion, we present a simple structure, high power 532 nm fiber laser system based on a 1064 nm YDFA and a highly efficient cavity-enhanced second-harmonic converter. Stable and reliable locking of the enhancement cavity is achieved with a piezoelectric ceramic transducer (PZT) actuator, which is controlled in a PDH scheme. Frequency doubling is achieved in a noncritically phase-matched LBO crystal. More than 30 W CW single-frequency 532 nm radiation with great beam quality is generated with an external conversion efficiency of 74%. The fiber-based single-frequency green laser is an efficient and reliable alternative pump source for a Ti-saphire laser, and may find applications in laser projects, laser shows, material processing, etc.
References
[1] K. V. Chellappan, E. Erden, H. Urey. Appl. Opt., 49, F79(2010).
[2] C. Chen, H. Chang, T. Chang, C. Chuang. Chin. Opt. Lett., 13, 110901(2015).
[3] H. J. Baving, H. Muuss, W. Skolaut. Appl. Phys. B, 29, 19(1982).
[4] J. Sakuma, Y. Asakawa, M. Obara. Opt. Lett., 29, 92(2004).
[5] S. Rabien, R. I. Davies, T. Ott, S. Hippler, U. Neumann. Proc. SPIE, 4494, 325(2002).
[6] O. A. Louchev, N. E. Yu, S. Kurimura, K. Kitamura. Appl. Phys. Lett., 87, 131101(2005).
[7] S. Spiekermann, F. Laurell, V. Pasiskevicius, H. Karlsson, I. Freitag. Appl. Phys. B, 79, 211(2004).
[8] S. Sinha, D. S. Hum, K. E. Urbanek, Y. W. Lee, M. J. F. Digonnet, M. M. Fejer, R. L. Byer. J. Lightwave Technol., 26, 3866(2008).
[9] Z. M. Liao, S. A. Payne, J. Dawson, A. Drobshoff, C. Ebbers, D. Pennington. J. Opt. Soc. Am. B, 21, 2191(2004).
[10] Y. H. Zheng, F. Q. Li, Y. J. Wang, K. S. Zhang, K. C. Peng. Opt. Commun., 283, 309(2010).
[11] L. McDonagh, R. Wallenstein. Opt. Lett., 32, 802(2007).
[12] Y. Z. Liu, Y. F. Cui, J. Zhang, A. M. Wang, Z. G. Zhang. Photon. Res., 3, 248(2015).
[13] M. A. Abdelalim, H. E. Kotb, H. Anis, S. Tchouragoulov. Photon. Res., 4, 277(2016).
[14] Q. Hao, W. X. Li, H. P. Zeng. Opt. Express, 17, 5815(2009).
[15] D. J. Richardson, J. Nilsson, W. A. Clarkson. J. Opt. Soc. Am. B, 27, B63(2010).
[16] R. Cieslak, W. A. Clarkson. Opt. Lett., 36, 1896(2011).
[17] T. Südmeyer, Y. Imai, H. Masuda, N. Eguchi, M. Saito, S. Kubota. Opt. Express, 16, 1546(2008).
[18] M. Stappel, R. Steinborn, D. Kolbe, J. Walz. Laser physics, 23, 1301(2012).
[19] T. Umeki, O. Tadanaga, M. Asobe. IEEE J. Quantum Electron., 46, 1206(2010).
[20] L. R. Taylor, Y. Feng, D. B. Calia. Opt. Express, 18, 8540(2010).
[21] A. Liu. Optics Express, 15, 977(2007).
[22] X. L. Wang, P. Zhou, H. Xiao, Y. X. Ma, X. J. Xu, Z. J. Liu. Laser Phys. Lett., 9, 591(2012).
[23] J. E. Rothenberg, P. A. Thielen, M. Wickham, C. P. Asman. Proc. SPIE, 6873, 68730O(2008).
[24] A. Ashkin, G. D. Boyd, J. M. Dziedzic. IEEE J. Quantum Electron., 2, 109(1966).