
- Photonics Research
- Vol. 9, Issue 2, 88 (2021)
Abstract
1. INTRODUCTION
Mode multiplexers/demultiplexers are widely used in space-division multiplexing (SDM) [1–5], free-space optical communication [6], coherent power combining [7–9], and wavefront analysis/synthesis [10]. One of the effective constructions of mode (de)multiplexers is based on multiplane light conversion (MPLC). So far, MPLC-based (de)multiplexers are mode selective (MS); each output mode is derived from only one input [11]. The transfer matrix representing the input–output relations for ideal MS (de)multiplexers is diagonal. For applications such as coherent power combining, in which multiple discrete input Gaussian beams are combined and transformed into a single beam essentially without power loss, an MS multiplexer simply cannot perform this function. To do so, one needs a non-mode-selective multiplexer (NMS mux) in which each output mode is a superposition of all inputs. Therefore, the transfer matrix for the NMS mux has nonzero off-diagonal elements [7]. Very recently, an MPLC-based NMS mux for coherent power combining was proposed [9]. However, that scheme only supported a few
In this paper, we present an approach to design MPLC-based NMS mux for HG modes. To generate each HG mode, the powers of all inputs remain the same while their phases form a Hadamard code. Furthermore, the Hadamard-coded inputs are arranged in a square array so that the symmetry and zero crossings in the input are the same as those of the corresponding output HG modes. This geometric structural similarity enables us to construct a 256 HG-mode NMS mux using only seven phase masks with performances comparable to those of MPLC-based MS multiplexers for HG modes.
2. MPLC BASED NON-MODE-SELECTIVE MODE MULTIPLEXER DESIGN
We illustrate our approach for a 16-mode NMS mux using Hadamard-coded [14] input beams arranged in a
Sign up for Photonics Research TOC. Get the latest issue of Photonics Research delivered right to you!Sign up now
Figure 1.Hadamard-coded MPLC-based NMS mux for 16 HG modes. (a) Input array; (b) the HG mode set that can be generated using this NMS mux; (c) the input array coded to produce (d) the desired output
Based on this approach, we need
For the given design, to generate pure HG modes, such as the
3. SIMULATION VERIFICATION
In our simulation, we modeled an MPLC-based NMS mux for 256 HG modes, with 256 inputs arranged in a
The seven calculated phase masks are shown in Fig. 2(a). The normalized intensities of the
Figure 2.(a) Calculated phase patterns for the seven phase masks used in the MPLC-based NMS mux supporting 256 HG modes; (b)–(d) normalized intensity of the
Figure 3 shows the input and output fields of some modes in comparison with the desired output ones. The output fields are very similar to the desired ones with identical zero-crossing structure and only minor differences, such as mode width and smoothness of lobes, indicating successful conversion of the inputs to the desired outputs. Identical zero-crossing structure is the dominant factor in determining the number of phase masks. All of the field images are zoomed in by a factor of approximately 3 for a better view.
Figure 3.Normalized field of some input (left column) and corresponding output (right column) modes generated by the MPLC-based NMS mux in comparison to the target mode (middle column).
To quantitatively evaluate the quality of output fields, we calculate the complex coupling coefficients as the overlap integral between the simulated output fields and the desired output fields. The power of the input and the desired output fields is normalized to 1. Figure 4(a) shows the squared magnitude of these coupling coefficients. The diagonal elements range from 0.45 to 0.56, demonstrating the high-level similarities between the actual output and desired output. The off-diagonal elements, ranging from
Figure 4.(a) Transfer matrix, (b) loss, and (c) modal crosstalk of the MPLC-based NMS mux supporting 256 HG modes.
Using the singular values
To further quantify the variation in the performance among all modes, we calculate the loss and modal crosstalk of each mode as follows:
The IL and MDL as functions of wavelength are plotted in Figs. 5(a) and 5(b), respectively. In the wavelength range from 1.50 to 1.60 μm, the IL and MDL vary about 1.7 dB and 5.1 dB, respectively. The larger variation of the MDL is attributed to the higher sensitivity to wavelength deviation of the mode with the minimum singular value, which contains high-spatial-frequency components. We observe that the bandwidth of the NMS muxes is narrower than that of MS muxes [16,17]. The underlying reason is that the phase masks for the NMS muxes have high-frequency components to match the aspect ratio difference between the input array and output HG modes.
Figure 5.(a) Insertion loss and (b) mode-dependent loss as functions of wavelength of the MPLC-based NMS mux supporting 256 HG modes.
4. CONTROL SCHEMES FOR COHERENT BEAM COMBINING: A COMPARISON
Our MPLC-based NMS mux can be used to generate pure HG modes, such as the
To demonstrate the advantage of phase-only control, we designed a 16-mode MPLC-based NMS mux with five phase masks with an area 1/16 of that for the 256-mode NMS mux to synthesize a fundamental-mode output. For phase-only control, the initial phases of the inputs are randomly set, while for phase-and-amplitude control, both phase and amplitude are randomly set. In both cases, the 16 inputs deviate from the desired state where the inputs have identical power and phase. The stochastic parallel-gradient-descent (SPGD) algorithm [18] was used to restore the inputs to the desired state by updating the phase/amplitude of the inputs until the power of synthesized beam within a predefined radius does not increase in five consecutive updates. This process is repeated 2000 times for different initial input states.
Figure 6 presents the results of the two control schemes for one of the 2000 initial states with the 16 amplitudes and phases in Figs. 6(c) and 6(f), respectively. The 16 inputs plotted in blue circles were initialized with the same random phases in the two control schemes; the amplitude in the amplitude-and-phase control scheme randomly deviates from 1 within the range between 0.5 and 2. The output beams before SPGD control have multiple speckles as shown in Figs. 6(a) and 6(d). After SPGD control, the output beams look more Gaussian, although some weak speckles remain as shown in Figs. 6(b) and 6(e). The output power for the phase-only control scheme is greater than for the amplitude-and-phase control scheme. This is evident in Figs. 6(c) and 6(f), where the amplitudes and phases of the 16 inputs after SPGD control are plotted using red squares. For the phase-only control scheme, the amplitude is always 1. In contrast, for the phase-and-amplitude control scheme, the amplitudes of some inputs drop below 1.
Figure 6.Comparison of (a)–(c) amplitude-and-phase control with (d)–(f) phase-only control by SPGD for coherent power combination with a NMS mux supporting 16 inputs. (a), (d) Output intensity before control; (b), (e) output intensity after control; (c), (f) the phase and amplitude of inputs before (blue) and after (red) control.
Better beam quality in terms of fidelity and faster convergence speed is also achieved with the phase-only control scheme. This can be seen by comparing the output power within a predefined radius equal to half width at half-maximum of the fundamental Gaussian beam, the power contained in the fundamental mode of the output beam, and the fidelity of the output beam as a function of SPGD iteration number shown in Figs. 7(a)–7(c), respectively. The results in Fig. 7 were the mean and error bars of the 2000 samples. Clearly, the phase-only control scheme has larger mean value and smaller variation throughout the adjustment process. From Figs. 7(d) and 7(e),the median of the normalized fundamental mode power for the phase-only control scheme was 0.70, outperforming the amplitude-and-phase control scheme, which had a median of 0.26. The reason for this difference is that amplitude control would reduce the power of some inputs out of phase relative to others [at opposite ends of diameters of the circles in Figs. 6(c) and 6(f)] to increase the total power in a defined aperture, whereas phase control tries to reduce the phase differences without attenuating amplitudes. In the amplitude-and-phase control scheme, the powers of the out-of-phase inputs usually cannot reduce to zero because of random perturbations and finite step sizes. The residual out-of-phase input introduces wavefront distortions and degrades the fundamental power. In contrast, there is no residual out-of-phase input in the phase control scheme, resulting in less distorted wavefront or stronger fundamental power. This difference is the reason why the fundamental power can be weaker than the power in the defined aperture in the amplitude-and-phase control scheme but always stronger in the phase control scheme, as well as the difference between the fundamental power histograms of the two control schemes.
Figure 7.Comparison between amplitude-and-phase control and phase-only control. (a) The average power of output beam in defined aperture and its error bar, (b) average fundamental mode power in the output beam and its error bar, and (c) average fidelity of the output beam and its error bar over 2000 samples as functions of adjustment times in the control process. Histogram of fundamental mode power in the output beam for 2000 samples for (d) amplitude-and-phase control and (e) phase-only control.
5. COMPARISON BETWEEN HADAMARD CODING AND FOURIER CODING
We considered another code set with all code elements having equal magnitude, such as the Fourier code set
To show the merit of Hadamard coding specifically, we compare the performance of two NMS muxes supporting 16 inputs, one coded by Hadamard code set and the other by Fourier code set. The Fourier coding is described by
Figure 8.Comparison between the optimized Fourier and randomly permutated Fourier coding schemes for an MPLC-based NMS mux for 16 inputs. (a) Coded phase of input array by the Hadamard coding (left), the optimized Fourier coding (middle), and a randomly permutated Fourier coding (right); matrix composed of the absolute values of the real part of the correlation coefficients between the Hadamard code set and (b) the optimized Fourier code set and (c) the randomly permutated Fourier code set; (d) MDL versus IL and (e) averaged total mode crosstalk versus IL of the 16-input NMS muxes using optimized Fourier coding (red star) and 1000 randomly permutated Fourier coding (blue circles).
Next, we compare the optimized Fourier coding with Hadamard coding by keeping the same simulation parameters. The IL, MDL, and total averaged modal crosstalk of the NMS muxes constructed by four to eight phase masks are plotted in Figs. 9(a)–9(c), respectively. Clearly, Hadamard coding has better performance than Fourier coding.
Figure 9.Comparison between the Fourier and Hadamard coding schemes for an MPLC-based NMS mux for 16 inputs in terms of (a) insertion loss, (b) mode-dependent loss, and (c) averaged total mode crosstalk with different numbers of phase masks.
The advantage of Hadamard coding is more obvious when increasing the number of supported modes. A similar comparison is carried out for a fixed number of phase masks (seven), but with a varying number of inputs and the same number of output HG modes, 16, 64, and 256. The results are shown in Figs. 10(a)–10(c). Clearly, the performance advantage of Hadamard coding in terms of IL and MDL becomes significant at 64 and 256 modes. The enlarged performance difference for a larger number of modes is due to the identical zero-crossing structure of the input array and output HG modes for Hadamard coding, whereas the correlation between the optimized Fourier and Hadamard code sets decreases. The results indicate that the Hadamard-coded NMS muxes are scalable with the number of modes, whereas the Fourier-coded ones are not.
Figure 10.Comparison between the Fourier and Hadamard coding schemes for an MPLC-based NMS mux with seven phase masks for different number of inputs in terms of (a) insertion loss, (b) mode-dependent loss, and (c) averaged total mode crosstalk.
6. APPLICATIONS IN BEAM SYNTHESIS
Another promising application of the NMS muxes is beam synthesis, in which an arbitrary beam is produced by controlling both the amplitude and phase of each element of the input array. This functionality, as a generalization of power combining, makes NMS muxes different from power combiners (in which only the fundamental mode is usually synthesized). Since the outputs of NMS muxes form a complete orthogonal mode base, an arbitrary beam can be decomposed into this mode base. The modal coefficients determine the amplitude and phase of each element of the input array as given by Eq. (3), where
In Fig. 11, we plot 16 synthesized Laguerre–Gaussian (LG) modes by the designed 256-mode NMS mux. The
Figure 11.Comparison of the synthesized and ideal LG modes and their corresponding interferogram resulting from interfering with a fundamental Gaussian mode with a positive wavefront curvature. The 16 LG modes belong to different mode groups with mode group numbers ranging from 0 to 15. The numbers in the figure are the squared magnitude of the coupling coefficients between the synthesized and corresponding ideal LG modes.
Different from HG mode generation, the power contribution from each input element to most LG modes is unequal, and therefore both amplitude control and phase control are needed. It is worth mentioning that the number of input elements with power contribution is larger than the required number of HG modes in the same mode group to synthesize a certain LG mode. Hence, the power of LG modes synthesized using a NMS mux is higher than by an MS mux, assuming that the power in the input element is equal.
7. CONCLUSION
An NMS mux based on MPLC for HG modes is designed and demonstrated by simulation. The Hadamard-coded input arrays are arranged with an identical zero-crossing structure to that of the desired HG modes, leading to a significant reduction in the number of required phase masks. Only seven phase masks were required for a 256-mode NMS mux. This device could be very useful for high-power coherent beam combining with phase-only control. Further increasing the number of supported modes is limited by the size and spatial resolution of the phase masks. As the number of modes goes up, the phase masks have to be enlarged to capture all the modes. The spatial resolution of the phase masks has to be increased accordingly to accommodate higher-spatial-frequency components contained in the higher-order modes. Phase masks with high resolution are hard to fabricate and pose difficulty in assembly and alignment. These limitations are common issues to all devices based on MPLCs, not uniquely associated with our proposed technique.
References
[1] R. Ryf, S. Randel, A. H. Gnauck, C. Bolle, R. Essiambre, P. J. Winzer, D. W. Peckham, A. McCurdy, R. Lingle. Space-division multiplexing over 10 km of three-mode fiber using coherent 6 × 6 MIMO processing. Optical Fiber Communication Conference and Exposition and National Fiber Optic Engineers Conference, 1-3(2011).
[2] E. Ip, N. Bai, Y. K. Huang, E. Mateo, F. Yaman, M. J. Li, S. Bickham, S. Ten, J. Liñares, C. Montero, V. Moreno, X. Prieto, V. Tse, K. M. Chung, A. Lau, H. Y. Tam, C. Lu, Y. Luo, G. D. Peng, G. Li. 88×3×112-Gb/s WDM transmission over 50 km of three-mode fiber with inline few mode fiber amplifier. 37th European Conference and Exposition on Optical Communications, Th.13.C.12(2011).
[4] H. Liu, H. Wen, J. C. A. Zacarias, J. E. Antonio-Lopez, N. Wang, P. Sillard, A. A. Correa, R. Amezcua-Correa, G. Li. 3×10 Gb/s mode group-multiplexed transmission over a 20 km few-mode fiber using photonic lanterns. Optical Fiber Communication Conference, M2D.5(2017).
[9] A. Billaud, F. Gomez, D. Allioux, N. Laurenchet, P. Jian, O. Pinel, G. Labroille. Optimal coherent beam combining based on multi-plane light conversion for high throughput optical feeder links. IEEE International Conference on Space Optical Systems and Applications (ICSOS), 1-5(2019).
[12] G. Arfken. Gram-Schmidt orthogonalization. Mathematical Methods for Physicists, 516-520(1985).
[13] J. Carpenter, N. K. Fontaine. Multi-plane light conversion with low plane count. Conference on Lasers and Electro-Optics Europe and European Quantum Electronics Conference (CLEO/Europe-EQEC)(2017).
[14] J. H. van Lint. Hadamard codes and generalizations. Introduction to Coding Theory, 47-48(1999).
[16] N. K. Fontaine, R. Ryf, H. Chen, D. Neilson, J. Carpenter. Design of high order mode-multiplexers using multiplane light conversion. European Conference on Optical Communication (ECOC), 1-3(2017).
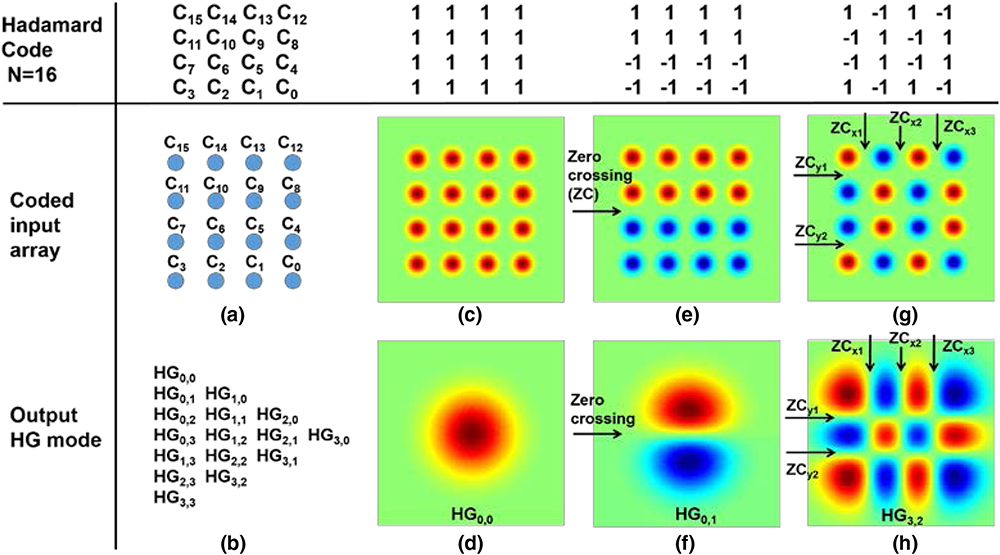
Set citation alerts for the article
Please enter your email address