
- Chinese Optics Letters
- Vol. 20, Issue 11, 112602 (2022)
Abstract
Keywords
1. Introduction
Ghost imaging (GI) is an imaging technique based on second-order intensity correlation[
The spatial resolution of a GI system is limited by the point spread function (PSF) of the system, just as in traditional imaging[
In this Letter, we put forward a novel scheme to enhance the resolution of GI by narrowing the PSF, referred to as second-order cumulants GI (SCGI). In our scheme, the fluctuation information of GI is exploited, which contains more information than traditional GI data and allows one to improve the resolution. We theoretically analyze the feasibility of the scheme and its performance in improving the resolution by second-order cumulants and experimentally verify results using a double-slit object. We also show that second-order cumulants can be used together with other modified GI schemes, such as CSGI, to obtain images with higher resolution.
Sign up for Chinese Optics Letters TOC. Get the latest issue of Chinese Optics Letters delivered right to you!Sign up now
2. Methods
A typical GI experimental setup is shown in Fig. 1, where a double-slit object is employed to assess the resolution[
Figure 1.Experimental setup to assess the resolution of GI. There is a digital micro-mirror device (DMD) in the source. The light illuminates the object and then is collected by a bucket detector (Dt) with no spatial resolution.
If
The intensity fluctuations correlation between
In order to minimize the imaging time, we consider the second-order cumulants, which can be written as
From Eqs. (9)–(11), we see that the intensity PSF of SCGI corresponds to a
Figure 2.PSF of a pinhole object at α′ = 0 for λ = 550 nm, z = 0.8 m, and R = 0.001 m by traditional GI (blue solid curve) and SCGI (red dash curve).
From Eq. (9), we see that
Figure 3.Image of the two-pinhole object by SCGI when α′ = −0.1 mm, α′′ = 0.1 mm, λ = 550 nm, R = 0.001 m, and z = 0.8 m: (a) L(α) = 0; (b) L(α) ≠ 0.
Compared to traditional GI, the resolution of SCGI improves even when
Second-order cumulants can also be used in other modified GI schemes, such as CSGI, which itself aims at improving the resolution of GI by reducing the effect of PSF on the information carried by
3. Results
We experimentally verify our theoretical predictions by using a computational GI setup. The light source is a projector (XE11F), and there is a digital mirror device (DMD) in the source. An optical spatial filter with a central wavelength of 550 nm is inserted in the light beam behind the projector. A bucket detector is composed of a lens and an optical detection circuit (LSSPD-2.5-3 P-08.26). The object is a double slit with width
First, we demonstrate that second-order cumulants can be used in traditional GI to enhance the resolution. In particular, we measure the resolution of traditional GI and traditional GI with
Figure 4.Experimental results for a double slit: (a)–(d) show results by traditional GI, SCGI [κ2(α) of traditional GI], CSGI, and SCGI [κ2(α) of CSGI], respectively.
We then verify that second-order cumulants can also be used in other modified GI schemes. In particular, with the same setup, we prove experimentally that
4. Discussion
In conclusion, we have used
References
[1] T. B. Pittman, Y. H. Shih, D. V. Strekalov, A. V. Sergienko. Optical imaging by means of two-photon quantum entanglement. Phys. Rev. A, 52, R3429(1995).
[2] C. Gao, X.-Q. Wang, L.-D. Gou, Y.-L. Feng, H.-J. Cai, Z-F. Wang, Z.-H. Yao. Ghost imaging for an occluded object. Laser Phys. Lett., 16, 065202(2019).
[3] C. Gao, X.-Q. Wang, Z.-F. Wang, Z. Li, G.-J. Du, F. Chang, Z.-H. Yao. Optimization of computational ghost imaging. Phys. Rev. A, 96, 023838(2017).
[4] A. Gatti, E. Brambilla, M. Bache, L. A. Lugiato. Ghost imaging with thermal light: comparing entanglement and classical correlation. Phys. Rev. Lett., 93, 093602(2004).
[5] P. H. S. Ribeiro, S. Pádua, J. C. Machado da Silva, G. A. Barbosa. Controlling the degree of visibility of Young’s fringes with photon coincidence measurements. Phys. Rev. A, 49, 4176(1994).
[6] A. Gatti, E. Brambilla, L. A. Lugiato. Entangled imaging and wave-particle duality: from the microscopic to the macroscopic realm. Phys. Rev. Lett., 90, 133603(2003).
[7] M. Ron, K. S. Deacon, Y. H. Shih. Ghost-imaging experiment by measuring reflected photons. Phys. Rev. A, 77, 041801(2008).
[8] J. Cheng, S.-H. Han. Incoherent coincidence imaging and its applicability in X-ray diffraction. Phys. Rev. Lett., 92, 093903(2004).
[9] A. F. Abouraddy, B. E. A. Saleh, A. V. Sergienko, M. C. Teich. Role of entanglement in two-photon imaging. Phys. Rev. Lett., 87, 123602(2001).
[10] E. Baleine, A. Dogariu, G. S. Agarwal. Correlated imaging with shaped spatially partially coherent light. Opt. Lett., 31, 2124(2006).
[11] X.-H. Chen, Q. Liu, K.-H. Luo, L.-A. Wu. Lensless ghost imaging with true thermal light. Opt. Lett., 34, 695(2009).
[12] M. O. Scully, M. S. Zubairy. Quantum Optics(1999).
[13] R. Hanbury Brown, R. Q. Twiss. LXXIV. A new type of interferometer for use in radio astronomy. Lond. Edinb. Dublin Philos. Mag. J. Sci., 45, 663(1954).
[14] R. Hanbury Brown, R. Q. Twiss. Correlation between photons in two coherent beams of light. Nature, 177, 27(1956).
[15] R. Hanbury Brown, R. Q. Twiss. A test of a new type of stellar interferometer on sirius. Nature, 178, 1046(1956).
[16] J. Cheng. Ghost imaging through turbulent atmosphere. Opt. Express, 17, 7916(2009).
[17] F. Ferri, D. Magatti, L. A. Lugiato, A. Gatti. Differential ghost imaging. Phys. Rev. Lett., 104, 253603(2010).
[18] X.-H. Chen, F.-H. Kong, Q. Fu, S.-Y. Meng, L.-A. Wu. Sub-Rayleigh resolution ghost imaging by spatial low-pass filtering. Opt. Lett., 42, 5290(2017).
[19] P. A. Moreau, E. Toninelli, P. A. Morris, R. S. Aspden, T. Gregory, G. Spalding, R. W. Boyd, M. J. Padgett. Resolution limits of quantum ghost imaging. Opt. Express, 26, 7528(2018).
[20] S.-Y. Meng, Y.-H. Sha, Q. Fu, Q.-Q. Bao, W.-W. Shi, G.-D. Li, X.-H. Chen, L.-A. Wu. Super-resolution imaging by anticorrelation of optical intensities. Opt. Lett., 43, 4759(2018).
[21] S. W. Hell, J. Wichmann. Breaking the diffraction resolution limit by stimulated emission: stimulated-emission-depletion fluorescence microscopy. Opt. Lett., 19, 780(1994).
[22] W. Larson, B. E. A. Saleh. Resurgence of Rayleigh’s curse in the presence of partial coherence. Optica, 5, 1382(2018).
[23] J. Du, W.-L. Gong, S.-S. Han. The influence of sparsity property of images on ghost imaging with thermal light. Opt. Lett., 37, 1067(2012).
[24] W.-L. Gong, S.-S. Han. Experimental investigation of the quality of lensless super-resolution ghost imaging via sparsity constraints. Phys. Lett. A, 376, 1519(2012).
[25] Y. Shechtman, S. Gazit, A. Szameit, Y. C. Eldar, M. Segev. Super-resolution and reconstruction of sparse images carried by incoherent light. Opt. Lett., 35, 1148(2010).
[26] J. Chen, W.-L. Gong, S.-S. Han. Sub-Rayleigh ghost imaging via sparsity constraints based on a digital micro-mirror device. Phys. Lett. A, 377, 1844(2013).
[27] X. Bai, Y.-Q. Li, S.-M. Zhao. Differential compressive correlated imaging. Acta. Phys. Sin., 62, 044209(2013).
[28] L.-Z. Li, X.-R. Yao, X.-F. Liu, W.-K. Yu, G.-J. Zhai. Super-resolution ghost imaging via compressed sensing. Acta. Phys. Sin., 63, 224201(2014).
[29] P.-L. Zhang, W.-L. Gong, X. Shen, D.-J. Huang, S.-S. Han. Improving resolution by the second-order correlation of light fields. Opt. Lett., 34, 1222(2009).
[30] J. Sprigg, T. Peng, Y. H. Shih. Super-resolution imaging using the spatial-frequency filtered intensity fluctuation correlation. Sci. Rep., 6, 38077(2016).
[31] K. Kuplicki, K. W. C. Chan. High-order ghost imaging using non-Rayleigh speckle sources. Opt. Express, 24, 26766(2016).
[32] Y.-L. Wang, Y.-N. Zhou, S.-X. Wang, F.-R. Wang, R.-F. Liu, H. Gao, P. Zhang, F.-L. Li. Enhancement of spatial resolution of ghost imaging via localizing and thresholding. Chin. Phys. B, 28, 044202(2019).
[33] Z.-S. Tong, Z.-T. Liu, C.-Y. Hu, J. Wang, S.-S. Han. Preconditioned deconvolution method for high-resolution ghost imaging. Photonics Res., 9, 1069(2021).
[34] W-X. Shi, C.-Y. Hu, S.-G. Yang, M.-H. Chen, H.-W. Chen. Optical random speckle encoding based on hybrid wavelength and phase modulation. Opt. Lett., 46, 3745(2021).
[35] F. Wang, C.-L. Wang, M.-L. Chen, W.-L. Gong, Y. Zhang, S.-S. Han, G. Situ. Far-field super-resolution ghost imaging with a deep neural network constraint. Light Sci. Appl., 11, 1(2022).
[36] H.-L. Ye, Y. Kang, J. Wang, L.-H. Zhang, H.-J. Sun, D.-W. Zhang. High resolution reconstruction method of ghost imaging via SURF-NSML. J. Korean Phys. Soc., 80, 964(2022).
[37] X. Zeng, Y.-F. Bai, X.-H. Shi, Y. Gao, X.-Q. Fu. The influence of the positive and negative defocusing on lensless ghost imaging. Opt. Commun., 382, 415(2017).
[38] F. Ferri, D. Magatti, A. Gatti, M. Bache, E. Brambilla, L. A. Lugiato. High-resolution ghost image and ghost diffraction experiments with thermal light. Phys. Rev. Lett., 94, 183602(2005).
[39] M. Paúr, B. Stoklasa, Z. Hradil, L. L. Sánchez-Soto, J. Rehacek. Achieving the ultimate optical resolution. Optica, 3, 1144(2016).
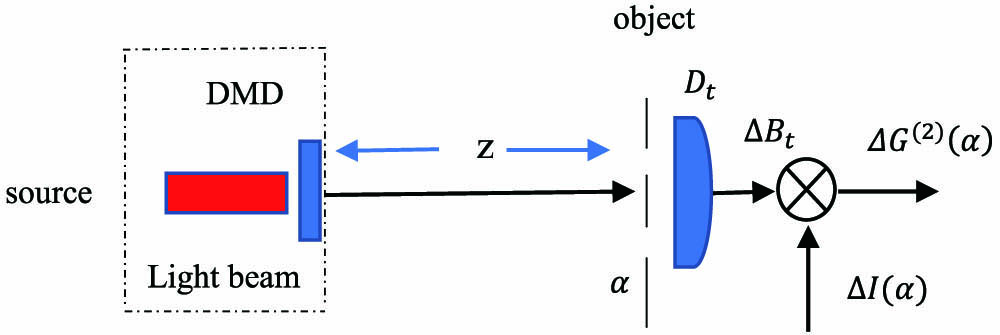
Set citation alerts for the article
Please enter your email address