
- Chinese Optics Letters
- Vol. 19, Issue 8, 081301 (2021)
Abstract
1. Introduction
Miniature laser devices that are constructed based on solid-state waveguide (WG) structures are gaining scientific and industrial interests owing to their hybrid functionality, highly compact platform, and inherent robustness. Moreover, benefiting from their small footprints, WG structures exhibit the ability of maintaining small spot sizes and hence high intra-cavity intensities, offering the attributes of reduced pump power threshold, high optical gain, and efficient lasing performance[
With proper optical pumping, visible light trace may appear in the crystal, especially in the case of
The past decade saw the tremendous progress of femtosecond (fs) laser inscription (FLI) as a flexible, versatile, and relatively low-cost technology for WG fabrication in a wide range of transparent optical materials[
Sign up for Chinese Optics Letters TOC. Get the latest issue of Chinese Optics Letters delivered right to you!Sign up now
In this work, we report on the fabrication of depressed cladding WGs in
2. Experimental Details
The surfaces and end-facets of the
To investigate the guiding behavior of the WGs, a linearly polarized 633 nm diode laser is coupled into the WG core regions through a typical end-face coupling arrangement. The propagation loss
A fiber-coupled confocal microscope (alpha300 R, WITec GmBH) is used to investigate the confocal micro-photoluminescence (µ-PL) properties of the fabricated WG1 as a representative. A 532 nm excitation laser is focused to a diffraction-limited spot size onto the WG cross section by using a
For laser characterization experiments, a Ti:sapphire cw laser (Coherent MBR 110, USA) generating a linearly polarized beam at 808 nm is employed as the pump source. A half-wave plate is used to control the polarization of the pump laser beam, so that the lasing properties in both transverse electric (TE) and transverse magnetic (TM) polarizations can be investigated. The pump beam is coupled into the WGs with a convex lens (
Similarly, the UC emission is excited with the polarized 808 nm Ti:sapphire laser. The same end-coupling system as for the WG laser characterization is utilized, except no cavity mirror is applied here.
3. Results and Discussion
Figure 1(a) shows the cross-sectional images of the produced WGs observed with an optical microscope. Zoomed-in images of WGs fabricated with 320 nJ pulse energy and with diameters of 35 µm (WG1), 30 µm (WG2), 25 µm (WG3), and 20 µm (WG4) are shown in Figs. 1(b)–1(e), which reveal distinct guiding boundaries without any cracks in the guiding cores or in the bulk. The intact cores are expected to preserve comparable optical properties to the substrate and, hence, possess potentials for laser generation.
Figure 1.(a) Microscopic image of the end-facet of the Nd,Gd:CaF2 sample with 16 cladding WGs deeply embedded beneath the surface. (b)–(e) The cross sections of WG1–WG4.
Figure 2(a) displays the output intensity (normalized) distributions measured under TM polarization. It is worthy to point out that these WGs could support arbitrary polarizations, and the mode distributions do not exhibit significant polarization dependence as a result of the symmetric morphology of the cladding structures. Strong optical confinements are obtained from all WGs. Particularly, single-mode guidance is observed from structures with a diameter of 20 µm due to the relatively small guiding areas. Furthermore, a WG produced with 200 nJ laser energy and 25 µm diameter, although possessing a larger size, also supports single-mode guidance owing to the low inscription energy that induces small RI modification. The propagation losses of the WG are shown in Fig. 2(b), which reveals that, in general, reduced propagation losses are obtained with larger guiding cores and higher irradiated laser energies. The minimum value is estimated to be around 0.87 dB/cm for WG1, with negligible differences for TE and TM polarizations. Hence, all of the results described hereafter are implemented with WG1. The RI contrast of WG1 is estimated to be around
Figure 2.(a) Mode intensity distributions from the fabricated cladding WGs at 633 nm under TM polarization. (b) Propagation losses of WGs obtained under both TM and TE polarizations.
Figure 3(a) shows the µ-PL emission spectra obtained from the unmodified bulk region, WG area, and laser-induced track, corresponding to the spots A, B, and C in the inset, respectively. It can be observed that, at the center of the damage track, the µ-PL intensity suffers from a strong quenching, while spectra from both WG and bulk are identical without any variance of spectral distribution and intensity. In order to obtain the detailed modification of µ-PL properties, the spatial dependences of the emitted intensity, peak position, and line width (FWHM) of the 867 nm line are investigated, as displayed in Figs. 3(b)–3(d), respectively. Meanwhile, for easy visualization and comparison, Figs. 3(e)–3(g) show the one-dimensional (1D) profiles corresponding to the positions indicated by the dashed lines in the inset of Fig. 3(a). It is clear that obvious intensity reduction in company with broadening of the emission line occurred at the filament locations, indicating high density of lattice defects and imperfections in these areas. In addition, blue shift of the µ-PL emission spectra in the filaments can be observed, which is aroused by lattice damages and expansive stress. These results coincide well with that obtained from WG structures in
Figure 3.(a) The room temperature µ-PL emission spectra of Nd3+ ions obtained from the WG area (blue), the filament (green), and the bulk of Nd,Gd:CaF2 crystals (red). The inset of (a) shows the cross section of WG1. The spatial two-dimensional (2D) distributions of (b) intensity, (c) peak shift, and (d) FWHM of 867 nm emission line obtained from the WG cross section. 1D distributions of (e) the emitted intensity, (f) energy shift, and (g) FWHM corresponding to the regions indicated by dashed lines in the inset of (a).
The laser output power from WG1 versus incident power is measured by using output couplers with 9% and 70% reflectance, the results of which are shown in Figs. 4(a) and 4(b). As can be seen, by using the 9% output coupler, the WG laser system exhibits a lasing threshold as low as 98.8 mW and 114.2 mW at TM and TE polarizations, respectively, while higher slope efficiency of around 13% is obtained with the 70% coupler, which leads to a maximum output power of 35 mW with 440 mW incident pump power, corresponding to an optical-to-optical conversion efficiency of around 8%. One can expect better lasing performance by further optimizing the WG structures and decreasing their propagation losses through, for example, increasing scan times for each track or enlarging the WG diameters. Figure 4(c) shows the generated WG laser spectrum, centered at 1059.91 nm with an FWHM of 6.7 nm. The inset of Fig. 4(c) demonstrates the lasing mode emitted from WG1, confirming the single-mode operation at 1.06 µm, which is an intriguing feature of this laser device for its applications.
Figure 4.cw WG laser output power as a function of the input pump power with (a) 9% and (b) 70% output coupler. (c) Spectrum of WG laser at 1059.91 nm. The inset shows the spatial intensity distribution of the output laser with a single-mode profile.
Figures 5(a) and 5(b) exhibit photographs of visible UC emissions observed in WG1 and the bulk area, from which strong confinement of luminescence in the WG can be observed, while clear intensity quenching occurred in the bulk. Figures 5(c) and 5(d) show the UC luminescence spectra obtained under increasing excitation power. When excited with the TE polarized laser, as shown in Fig. 5(a), the luminescence consists of a short-wavelength range (SWR) from 415 nm to 550 nm and a long-wavelength range (LWR) of 550 nm to 625 nm, with each waveband splitting into several subpeaks. In contrast, with TM polarized laser excitation, the wavelength regions preserve the original positions, whereas LWR is nearly absent, leading to a large intensity ratio of SWR and LWR components. A key feature of the spectrum, under both TE and TM polarized laser excitations, is the steady increase of the luminescence emission with the increasing excitation power.
Figure 5.Photographs of visible UC luminescence generated from (a) WG1 and (b) the bulk. UC spectra obtained from WG1 under (c) TE and (d) TM polarizations with varying excitation powers.
In order to investigate the effect of polarization of the excitation laser on the UC, the generated luminescence spectra are measured as the half-wave plate rotating from angles of 0° to 90° under the same coupling conditions, corresponding to polarization varying from TE to TM and then back to TE. The results are shown in Fig.?6(a), further confirming the strong polarization dependence of the luminescence spectra. To get a clear picture of the competition between SWR and LWR, we focus on two stationary peaks at 485?nm and 585?nm. In Figs.?6(b) and 6(c), the experimentally determined luminescence intensities are shown as a function of the angles of the polarizer, which demonstrate strong periodic dependence of each peak on the polarization. The intensity ratios between the two peaks are determined and presented in Figs.?6(d) and 6(e). The maximum values of the spectral intensity ratio of I485/I585 and I585/I485are found to be 12.16 and 4.76, respectively. The variation of UC spectra, in a first-order approximation, can be interpreted from the perspective of ESA, whose cross sections show polarized difference in
Figure 6.(a) UC spectra from WG1 obtained with a rotating half-wave plate. Luminescence intensities of emission lines centered at (b) 485 nm and (c) 585 nm as a function of the angles of the polarizer. The intensity ratios of (d) I485/I585 and (e) I585/I485 as a function of the angles of the polarizer.
4. Conclusion
Cladding WGs are fabricated in a
References
[1] C. Grivas. Optically pumped planar waveguide lasers: part II: gain media, laser systems, and applications. Prog. Quantum Electron., 45–46, 3(2016).
[2] Y. C. Jia, F. Chen. Compact solid-state waveguide lasers operating in the pulsed regime: a review. Chin. Opt. Lett., 17, 012302(2019).
[3] F. Chen, J. R. Vázquez de Aldana. Optical waveguides in crystalline dielectric materials produced by femtosecond-laser micromachining. Laser Photon. Rev., 8, 251(2014).
[4] F. Chen. Micro- and submicrometric waveguiding structures in optical crystals produced by ion beams for photonic applications. Laser Photon. Rev., 6, 622(2012).
[5] J. Leute, N. Huntemann, B. Lipphardt, C. Tamm, P. B. R. Nisbet-Jones, S. A. King, R. M. Godun, J. M. Jones, H. S. Margolis, P. B. Whibberley, A. Wallin, M. Merimaa, P. Gill, E. Peik. Frequency comparison of 171Yb+ ion optical clocks at PTB and NPL via GPS PPP. IEEE. Trans. Ultrason. Ferr., 63, 981(2016).
[6] C. Kränkel, D. T. Marzahl, F. Moglia, G. Huber, P. W. Metz. Out of the blue: semiconductor laser pumped visible rare-earth doped lasers. Laser Photon. Rev., 10, 548(2016).
[7] Y. Y. Ren, C. Cheng, Y. C. Jia, Y. Jiao, D. W. Li, M. D. Mackenzie, A. K. Kar, F. Chen. Switchable single-dual-wavelength Yb,Na:CaF2 waveguide lasers operating in continuous-wave and pulsed regimes. Opt. Mater. Express, 8, 1633(2018).
[8] C. Grivas, C. Corbari, G. Brambilla. Recent progress in continuous-wave Ti:sapphire waveguide lasers. Proc. SPIE, 8988, 898808(2014).
[9] Y. Y. Ren, G. Brown, R. Mary, G. Demetriou, D. Popa, F. Torrisi, A. C. Ferrari, F. Chen, A. K. Kar. 7.8-GHz graphene-based 2-µm monolithic waveguide laser. IEEE. J. Set. Top. Quantum Electron., 21, 1602106(2015).
[10] S. A. Mcdaniel, A. Lancaster, J. W. Evans, A. K. Kar, G. Cook. Power scaling of ultrafast laser inscribed waveguide lasers in chromium and iron doped zinc selenide. Opt. Express, 24, 3502(2016).
[11] J. E. Moffact, G. Tsiminis, E. Klantsataya, T. J. de Prinse, D. Ottaway, N. A. Spooner. A practical review of shorter than excitation wavelength light emission processes. Appl. Spectrosc. Rev., 55, 327(2019).
[12] W. J. Yao, Q. Y. Tian, W. Wu. Tunable emissions of upconversion fluorescence for security applications. Adv. Opt. Mater., 7, 1801171(2019).
[13] D. Y. Li, H. Ågren, G. Y. Chen. Near infrared harvesting dye-sensitized solar cells enabled by rare-earth upconversion materials. Dalton. Trans., 47, 8526(2018).
[14] Y. Qiao, S. H. Li, W. H. Liu, M. Q. Ran, H. F. Lu, Y. P. Yang. Recent advances of rare-earth ion doped luminescent nanomaterials in perovskite solar cells. Nanomaterials, 8, 43(2018).
[15] Y. Choi, S. H. Baek, S. J. Chang, Y. Song, R. Rafique, K. T. Lee. Synthesis of upconversion nanoparticles conjugated with graphene oxide quantum dots and their use against cancer cell imaging and photodynamic therapy. Biosens. Bioelectron., 93, 267(2017).
[16] A. Fernandez-Bravo, K. Y. Yao, E. S. Barnard, N. J. Borys, E. S. Levy, B. Tian, C. A. Tajon, L. Moretti, M. V. Altoe, S. Aloni, K. Beketayev, F. Scotognella, B. E. Cohen, E. M. Chan, P. J. Schuck. Continuous-wave upconverting nanoparticle microlasers. Nat. Nanotechnol., 13, 572(2018).
[17] B. Guzelturk, Y. Kelestemur, K. Gungor, A. Yeltik, M. Z. Akgul, Y. Wang, R. Chen, C. Dang, H. Sun, H. V. Demir. Stable and low-threshold optical gain in CdSe/CdS quantum dots: an all colloidal frequency upconverted laser. Adv. Mater., 27, 2741(2015).
[18] Z. B. Li, J. X. Yang, M. Shi, L. Yang. Upconversion luminescence of graphene oxide through hybrid waveguide. J. Phys. Chem. C, 122, 16866(2018).
[19] J. M. Lv, X. T. Hao, F. Chen. Green up-conversion and near-infrared luminescence of femtosecond-laser-written waveguides in Er3+, MgO co-doped nearly stoichiometric LiNbO3 crystal. Opt. Express, 24, 25482(2016).
[20] L. M. Zhang, T. Y. Guo, Y. Y. Ren, Y. J. Cai, M. D. Mackenzie, A. K. Kar, Y. C. Yao. Cooperative up-converted luminescence in Yb,Na:CaF2 cladding waveguides by femtosecond laser inscription. Opt. Commun., 441, 8(2019).
[21] R. Osellame, H. Hoekstra, G. Cerullo, M. Pollnau. Femtosecond laser microstructuring: an enabling tool for optofluidic lab-on-chips. Laser Photon. Rev., 5, 442(2011).
[22] D. Choudhury, J. R. Macdonald, A. K. Kar. Ultrafast laser inscription: perspectives on future integrated applications. Laser Photon. Rev., 8, 827(2014).
[23] K. Sugioka, Y. Cheng. Ultrafast lasers-reliable tools for advanced materials processing. Light Sci. Appl., 3, e149(2014).
[24] D. Z. Tan, K. N. Sharafudeen, Y. Z. Yue, J. R. Qiu. Femtosecond laser induced phenomena in transparent solid materials: fundamentals and applications. Prog. Mater. Sci., 76, 154(2016).
[25] A. G. Okhrimchuk, A. V. Shestakov, I. Khrushchev, J. Mitchell. Depressed cladding, buried waveguide laser formed in a YAG: Nd3+crystal by femtosecond laser writing. Opt. Lett., 30, 2248(2005).
[26] Y. Y. Ren, G. Brown, A. Ródenas, S. Beecher, F. Chen, A. K. Kar. Mid-infrared waveguide lasers in rare-earth-doped YAG. Opt. Lett., 37, 3339(2012).
[27] Y. C. Jia, R. Y. He, J. R. Vázquez de Aldana, H. L. Liu, F. Chen. Femtosecond laser direct writing of few-mode depressed-cladding waveguide lasers. Opt. Express, 27, 30941(2019).
[28] Y. Y. Ren, F. Chen, J. R. Vázquez de Aldana. Near-infrared lasers and self-frequency-doubling in Nd:YCOB cladding waveguides. Opt. Express, 21, 11562(2013).
[29] J. Du, X. Y. Liang, Y. G. Wang, L. B. Su, W. W. Feng, E. W. Dai, Z. Z. Xu, J. Xu. 1 ps passively mode-locked laser operation of Na,Yb:CaF2 crystal. Opt. Express, 13, 7970(2005).
[30] L. B. Su, J. Xu, H. J. Li, W. Q. Yang, Z. Q. Zhao, J. L. Si, Y. J. Dong, G. Q. Zhou. Codoping Na+ to modulate the spectroscopy and photoluminescence properties of Yb3+ in CaF2 laser crystal. Opt. Lett., 30, 1003(2005).
[31] J. L. Doualan, L. B. Su, G. Brasse, A. Benayad, V. Menard, Y. Y. Zhan, A. Braud, P. Camy, J. Xu, R. Moncorgé. Improvement of infrared laser properties of Nd:CaF2 crystals via codoping with Y3+ and Lu3+ buffer ions. J. Opt. Soc. Amer. B, 30, 3018(2013).
[32] S. Y. Pang, F. K. Ma, H. Yu, X. B. Qian, D. P. Jiang, Y. J. Wu, F. Zhang, J. Liu, J. Y. Xu, L. B. Su. Highly efficient continuous-wave laser operation of LD-pumped Nd,Gd:CaF2 and Nd,Y:CaF2 crystals. Laser Phys. Lett., 15, 055802(2018).
[33] T. Y. Guo, R. N. Li, L. F. Sun, Y. J. Cai, Y. Y. Ren, Y. C. Yao, M. D. Mackenzie, A. K. Kar. Femtosecond laser inscribed Pr:CaF2 waveguides: micro-spectroscopy characterizations and refractive index reconstruction. Opt. Commun., 461, 125243(2020).
[34] Y. Guyot, H. Manaa, J. Y. Rivoire, R. Moncorgé, N. Garnier, E. Descroix, M. Bon, P. Laporte. Excited-state-absorption and upconversion studies of Nd3+-doped single crystals Y3Al5O12, YLiF4, and LaMgAl11O19. Phys. Rev. B, 51, 784(1995).
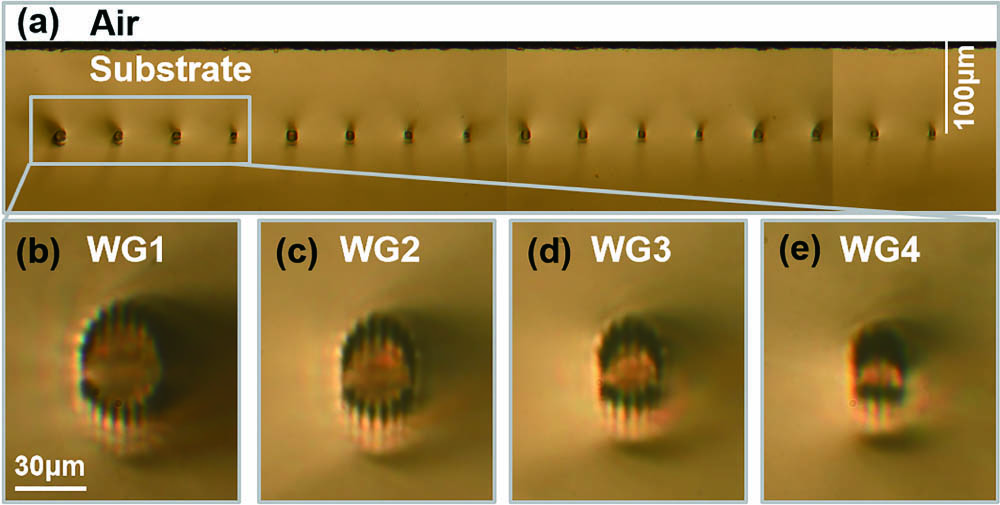
Set citation alerts for the article
Please enter your email address