P. Bradford1、*, M. P. Read1, M. Ehret2, L. Antonelli1, M. Khan1, N. Booth3, K. Glize3, D. Carroll3, R. J. Clarke3, R. Heathcote3, S. Ryazantsev4, S. Pikuz4, C. Spindloe3, J. D. Moody5, B. B. Pollock5, V. T. Tikhonchuk6, C. P. Ridgers1, J. J. Santos7, and N. C. Woolsey1
Author Affiliations
1York Plasma Institute, Department of Physics, University of York, Heslington, York YO10 5DD, UK2Centre Lasers Intenses et Applications, University of Bordeaux-CNRS-CEA, 33405 Talence, France3Central Laser Facility, Rutherford Appleton Laboratory, Chilton, Didcot, STFC, UKRI, Oxfordshire, UK4Joint Institute for High Temperatures, RAS, Moscow125412, Russia5Lawrence Livermore National Laboratory, Livermore, California 94551, USA6Centre Lasers Intenses et Applications, University of Bordeaux-CNRS-CEA, 33405 Talence, France7Centre Lasers Intenses et Applications, University of Bordeaux-CNRS-CEA, 33405 Talence, Franceshow less
Fig. 1. Left: Sample proton radiograph taken perpendicularly to the axis of a 2-mm-diameter wire loop with
$E_{p}=7.3\pm 0.05~\text{MeV}$ protons. The void width,
$w$, is proportional to the square root of the current flowing in the coil loop
[17], though
$w$ is also affected by electric fields. Right: Sample proton radiograph taken parallel to the axis of a 1-mm-diameter wire loop with
$E_{p}=6.5\pm 0.07~\text{MeV}$ protons. Notice how the outline of an Au grid has been imprinted in the proton beam as a fiducial. Each RCF image has a magnification of
$M=10$, so a distance of 5 mm in the detector plane (indicated above) equates to 0.5 mm in the coil plane.
Fig. 2. Photograph of full capacitor coil target assembly with two proton foils and Au grids. Two rectangular Au foils of $40~\unicode[STIX]{x03BC}\text{m}$ thickness with $5~\unicode[STIX]{x03BC}\text{m}$ Au shields were used for TNSA proton radiography. Between the proton foils and the capacitor coil, two Au grids were installed to act as visual references in the proton images. RCF stacks were positioned 10 cm behind the target to detect the protons along two axes.
Fig. 3. Comparison of EPOCH simulations with RCF data for (a) 1-mm- and (b) 2-mm-diameter capacitor coil loops. EPOCH simulations used a monoenergetic $E_{p}=7~\text{MeV}$ proton beam with a divergence angle of $40^{\circ }$. The RCF data shown corresponds to protons with energy $E_{p}=7.3\pm 0.05~\text{MeV}$. Proton voids are bigger at early times ($t\sim 0.3~\text{ns}$) before decaying to a stable value for $t>0.8~\text{ns}$. Estimated loop currents at $t>0.8~\text{ns}$ are $J=5~\text{kA}$ for both loop diameters. The magnification of each RCF image is $M=10$, so a distance of 5 mm in the detector plane (indicated above) equates to 0.5 mm in the coil plane.
Fig. 4. Left: Experimental RCF data for a 1-mm-diameter loop taken at $t\sim 0.8~\text{ns}$ with $E_{p}=7.3\pm 0.05~\text{MeV}$ protons. Right: Synthetic proton radiograph for 7 MeV protons passing across a 1-mm-diameter loop with capacitor-coil-shaped B-field and a uniformly charged circular ring. Note that the loop current that best matches the RCF data is now three times higher than that in Figure 3 ($J=15~\text{kA}$ versus $J=5~\text{kA}$). Horizontal and vertical lines are provided as fiducials. The magnification of each RCF image is $M=10$, so a distance of 5 mm in the detector plane (indicated above) equates to 0.5 mm in the coil plane.
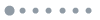
Fig. 5. Demonstration of the effect of positive wire electric fields on proton void structure. In these deflectometry simulations, 7 MeV protons were propagated perpendicularly across a 1-mm-diameter wire loop. Horizontal and vertical lines have been cut out of the proton distribution to act as fiducials. Left: Simulation run with electric fields only. Electric fields were calculated for a uniformly charged wire loop with total charge $Q=10~\text{nC}$. Proton displacement is approximately constant across the entire length of the wire. Distortion of the fiducial grid is only observed near the top of the loop – not near the vertical wire sections. Right: Simulation run with electric and magnetic fields. Electric fields were calculated for a uniformly charged wire loop with total charge $Q=5~\text{nC}$, while magnetic fields were generated from a uniform wire current of $J=5~\text{kA}$. The proton void width is 6 mm – approximately 1 mm larger than that observed for the same simulation without an electric field.
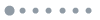
Fig. 6. (a) Axial proton radiograph for a 2-mm-diameter loop, $t\sim 0.8~\text{ns}$ after the beginning of the laser drive. Image taken using $E_{p}=7.3\pm 0.05~\text{MeV}$ protons. Two areas have been highlighted with white circles: Region 1, axial proton void at the centre of the capacitor coil loop; Region 2, grid distortion is concentrated at the base of the vertical wires and around the plates. (b) Synthetic proton radiograph for a circular ring of current ($J=40~\text{kA}$) with an overlapped circular ring of charge ($Q=-80~\text{nC}$). (c) Synthetic proton radiograph for a capacitor coil wire carrying $J=40~\text{kA}$ with an overlapped uniform charge distribution ($Q=-110~\text{nC}$). Each RCF image is magnified by a factor $M=10$, so a distance of 5 mm in the detector plane (indicated above) equates to 0.5 mm in the coil plane. Insets in the bottom right hand corner of (b) and (c) are diagrams of the conductor geometry used in each simulation.
Fig. 7. Synthetic proton radiographs for a 1-mm-diameter charged ring on top of a capacitor coil loop carrying a current $J=15~\text{kA}$. Vertical and horizontal lines have been cut out of the proton beam to act as fiducials. (a) Grid deflection is minimal around the loop for B-field simulations with a static current. (b) For $Q=-10~\text{nC}$, we see millimetre-scale grid deflections consistent with the RCF data. (c) For $Q=-40~\text{nC}$, grid deflection is of centimetre scale and much larger than that observed on the RCF.
Fig. 8. (a) Synthetic radiograph for a 7 MeV divergent proton beam passing through the magnetic field of a 2-mm-diameter current loop carrying a current $J=100~\text{kA}$. A vertical slot is cut out of the Gaussian proton distribution, which rotates through an approximately fixed $13^{\circ }$ angle inside the loop. (b) Blue points: graph of loop current plotted against rotation angle of the fiducial grid for a 2-mm-diameter current loop. Since there is no evidence of grid rotation in the RCF data, this puts an upper limit on the loop current of less than $J=10~\text{kA}$. The straight line represents proton gyration angle for protons passing perpendicularly through a uniform magnetic field of 1 mm spatial scale. The magnitude of this magnetic field is equivalent to the B-field at the centre of a 2-mm-diameter current loop.
Fig. 9. (a) Study of grid rotation with different loop charges. Negative charge is distributed uniformly along the capacitor coil loop and the proton beam divergence angle is fixed at $40^{\circ }$. We can see that the angle of rotation of the grid is unchanged for different loop charges. (b) Proton beam with zero divergence passing through the magnetic field of a current loop carrying $J=40~\text{kA}$ – the grid rotation angle is unchanged versus the $40^{\circ }$ case.
Fig. 10. (a) Magnetic field geometry used in simulations of two vertical wires with opposite currents. (b) Synthetic radiograph for two vertical wires carrying $J=\pm 20~\text{kA}$. Horizontal fiducial demonstrates multi-millimetre grid deflection close to the wire surface. Approximate location of the wire surface is picked out with vertical dashed lines. (c) Detail from RCF image of 1-mm-diameter loop taken $t\sim 0.8~\text{ns}$ after the beginning of the laser drive. Though there is no clear evidence of a continuous grid deflection around the vertical wires, a smeared-out region ${\sim}1{-}2~\text{mm}$ thick around the wire puts an upper limit on the wire current at $J\sim 5~\text{kA}$.