
- High Power Laser Science and Engineering
- Vol. 10, Issue 1, 010000e4 (2022)
Abstract
Keywords
1 Introduction
High-brightness, highly stable and fully coherent pulses from high repetition rate free-electron lasers (FELs) in the extreme ultraviolet (EUV) and X-ray spectral regimes are capable of driving scientific applications[1,2] such as time-resolved coherent spectroscopy[3], photon scattering[4] and coherent control[5,6]. Currently available megahertz repetition rate short-wavelength FEL user facilities, the European XFEL[7] and FLASH[8], both operate in burst mode. Several continuous wave (CW) facilities are under construction, including the LCLS-II[9] and SHINE[10]. One of the main operation modes for these FEL facilities is self-amplified spontaneous emission (SASE)[11,12]. Originating from electron shot noise, SASE FELs have poor temporal coherence and large shot-to-shot fluctuations[13].
For the current FEL facilities worldwide[12,14], one effective method to improve the temporal coherence is adopting seeded schemes, which can be classified as self-seeding and external seeding. Compared with self-seeding schemes, external seeding schemes, including high-gain harmonic generation (HGHG)[15–17] and echo-enabled harmonic generation (EEHG)[18–21], can generate FEL pulses with lower energy fluctuation. They also provide extra capabilities to be highly synchronized with the external laser and to control the coherence in multi-color, multi-pulse implementations[6,22]. These fulfill the requirements of the aforementioned scientific applications.
In HGHG FELs[15], a sinusoidal energy modulation is first introduced to the electrons via the interaction between the electron beam and an external seed laser within a modulation undulator (called a modulator). After a magnetic chicane, the energy modulation is converted to density modulation, creating micro-bunching at the harmonic of the seed laser wavelength. The bunched beam is then delivered to a radiation undulator (called a radiator) resonant at the harmonic wavelength. To obtain sufficient bunching at a higher harmonic, the amplitude of the energy modulation imprinted by the seed laser needs to be large enough. However, for efficient FEL amplification in the radiator, the induced electron beam energy spread should be kept at a low level. This, in turn, places a restriction on the highest achievable harmonic for an HGHG FEL. For example, for the FERMI FEL-1, the highest harmonic for FEL emission was the 13th harmonic, although coherent emission could be observed at a higher (15th) harmonic[17]. Recently, the operation range of the FERMI FEL-1 was extended to the 25th harmonic by employing an electron beam with a low slice energy spread[23].
Sign up for High Power Laser Science and Engineering TOC. Get the latest issue of High Power Laser Science and Engineering delivered right to you!Sign up now
For high repetition rate HGHG FELs, the external laser becomes a determinant at the megahertz repetition rate. The state-of-the-art laser system capable of delivering the hundred-megawatt pulses required by an HGHG scheme is limited to the kilohertz repetition rate. Several methods have been proposed to relax the peak power or repetition rate requirements for the seed laser[24], including using an optical cavity for seed laser recirculation and/or amplification[25,26] and employing a long modulator for both seed laser amplification and electron beam modulation[27–29]. To enhance the energy modulation efficiency, a self-modulation HGHG scheme was also proposed[27,30], which was demonstrated at the Shanghai Soft X-ray Free-electron Laser facility (SXFEL) recently[31,32]. With a single-stage HGHG setup, the seventh harmonic emission has been obtained, while with a two-stage cascaded HGHG setup and the ‘fresh bunch’ technique, the 30th harmonic emission has been obtained. It is worth noting that the self-modulator was also proposed to resonate at the second harmonic of the seed laser wavelength in Refs. [27,31], in which case the 10th harmonic emission could be obtained according to simulation.
In this paper, we study a compact harmonic-enhanced HGHG scheme to further extend the highest harmonic for FEL emission in a high repetition rate, single-stage HGHG scheme. Compared to a regular HGHG scheme, an additional modulation undulator resonant at a high-harmonic wavelength is introduced, which essentially causes efficient electron density modulation up to the 20th harmonic wavelength, but with a relaxed peak power requirement for the seed laser and reduced electron energy spread growth. Moreover, since the electrons are bunched at a high harmonic of the seed laser wavelength, the density modulation can be achieved via a short dispersion section. This ensures the compactness of the proposed scheme.
The remainder of this paper is organized as follows. In Section 2, we give a detailed description of the scheme. In Section 3, the performance of the scheme is illustrated with simulations. Section 4 gives a summary and some discussions.
2 The scheme
A layout of the proposed scheme is sketched in Figure 1, which mainly comprises a short modulation undulator
Figure 1.Schematic layout of the harmonic-enhanced HGHG FEL. Here, is a short modulation undulator, where an external seed laser with a wavelength of
and a peak power at the megawatt level is used to modulate the electron beam;
is a harmonic-enhanced modulation undulator resonant at
and
is a radiation undulator resonant at
;
and
are two dispersion chicanes. (a)–(e) Sketches of electron beam distribution in the longitudinal phase space.
A similar layout can be found in the scheme of self-modulation HGHG[31], where the first stage of the HGHG radiator is resonant at the fundamental or the second harmonic wavelength. Our work can be distinguished by the high-order harmonic modulator and the significant bunching factor up to the 20th harmonic of the seed laser wavelength. Another scheme with a similar layout is the double-stage harmonic cascade HGHG demonstrated at the FERMI FEL to extend the wavelength coverage of the HGHG scheme[33]. However, the first-stage radiator resonates at a much higher harmonic (13th) wavelength, which then requires a higher seed laser power for the high repetition rate operation.
For sufficient FEL emission at harmonic wavelength, it is essential to induce effective electron bunching at the target wavelength while keeping the energy spread growth as low as possible. In this scheme, the electron beam undergoes two-stage modulation, one in the fundamental modulator
The setup of the scheme can be very compact. Since the optimal dispersion of
3 FEL simulations of harmonic-enhanced HGHG
To demonstrate the feasibility of the proposed scheme, time-dependent FEL simulations using GENESIS code[37] have been carried out, with the representative parameters summarized in Table 1. The electron beam was assumed to have a central energy of 1 GeV, a slice energy spread (
Parameter | Value | Unit |
---|---|---|
Energy | 1 | GeV |
Energy spread | 0.1 | MeV |
Current | 800 | A |
Emittance | 1 | mm |
Bunch charge | 100 | pC |
Wavelength | 270 | nm |
Peak power | 1 | MW |
Pulse length | 150 | fs |
8 | cm | |
3.2 | m | |
4 | cm | |
3 | m |
Table 1. Main parameters used in the simulations.
For the proposed scheme, a proper choice of the harmonic number
Similarly, the length of the harmonic-enhanced modulator should also be chosen properly. On one hand,
In this case, the interaction between the seed laser and the electron beam in
Figure 2.Electron distribution in the longitudinal phase space and the corresponding bunching factor at different harmonics of the seed laser wavelength after (a), (b) , (c), (d)
and (e), (f)
.
The longitudinal phase space after
To fully convert the electron energy modulation after
Fifty GENESIS runs were then performed with different random shot noise initializations and with the radiator resonant at the wavelength of 13.5 nm (
Figure 3.(a) The evolution of radiation pulse energy along and
averaged over 50 GENESIS runs and (b) the FEL spectra for the 50 runs for the case with
. The averaged spectrum is plotted as a dark line in (b).
To facilitate the estimation of repetition rate improvement of the proposed scheme compared to a regular HGHG scheme, we simulated a regular HGHG scheme using the same parameters for the modulator
We also investigated the case without
Figure 4.Bunching factor evolution at the fifth and 20th harmonics of seed laser wavelength along the radiation undulator for the case without
.
The evolutions of the radiation spectrum, electron phase space and beam current profile along
Figure 5.Evolution of the radiation spectra (upper) and the longitudinal phase space of the electron beam (lower) along : (a) and (e) at the entrance of
, (b) and (f) after the first undulator segment, (c) and (g) after the third segment and (d) and (h) after the fifth segment. The longitudinal coordinates are scaled to
. The electron current profiles are plotted as red curves in (e)–(h).
Fifty GENESIS runs have been performed as well, and the results are plotted in Figure 6. As shown in Figure 6(a), the FEL saturates at 21.6 m of
Figure 6.(a) The evolution of radiation pulse energy along and
averaged over 50 GENESIS runs and (b) the FEL spectra for the 50 runs for the case without
. The averaged spectrum is plotted as a dark line in (b).
We finally investigated the robustness of the scheme against machine errors. For the simulation, we used the configuration without
Figure 7.FEL pulse energy for 200 GENESIS runs with random machine errors.
The radiation bandwidth of the proposed scheme is also sensitive to the nonlinear energy chirp, as is a normal HGHG scheme. Some methods have been developed to control the nonlinear energy chirp at a low level[42]. With an assumed 0.3 MeV/
4 Summary and discussion
In summary, we have studied a robust harmonic-enhanced HGHG scheme to generate high repetition rate coherent FEL using a low peak power seed laser. The performance for two possible configurations has been investigated using GENESIS simulations, one with a short dispersion chicane after the harmonic-enhanced modulator and the other without the chicane. Both configurations can generate coherent FEL output at 13.5 nm with a 270 nm, 1 MW (peak power) seed laser. For the case with the dispersion chicane, a bunching factor of 0.13 can be achieved at the 20th harmonic of the seed laser wavelength before the electron beam enters the radiator, and the FEL saturates at about 13.6 m in the radiator with a pulse energy of 193 μJ. The case without the dispersion chicane has a longer saturation length of 21.6 m and a lower pulse energy of 176 μJ. However, it is only necessary to tune a few radiation undulator segments in a regular HGHG scheme for it to be resonant at a higher harmonic (e.g., fifth harmonic in our simulation) of the fundamental wavelength. The length of these undulator segments (i.e., the harmonic-enhanced modulator) can be varied easily, providing the flexibility of optimizing the harmonic-enhanced modulator for different harmonics. In particular, with a longer modulator, even higher harmonics for soft X-ray emission might be reached. Besides, a few methods can be used to further improve the FEL performance. For example, the first undulator segment of
References
[1] E. A. Seddon, J. A. Clarke, D. J. Dunning, C. Masciovecchio, C. J. Milne, F. Parmigiani, D. Rugg, J. C. H. Spence, N. R. Thompson, K. Ueda, S. M. Vinko, J. S. Wark, W. Wurth. Rep. Prog. Phys., 80, 115901(2017).
[2] N. Huang, H. Deng, B. Liu, D. Wang, Z. Zhao. Innovation, 2, 2(2021).
[3] M. Dell’Angela, F. Hieke, F. Sorgenfrei, N. Gerken, M. Beye, N. Gerasimova, H. Redlin, W. Wurth. Surf. Sci., 643, 197(2016).
[4] R. R. Fäustlin, T. Bornath, T. Döppner, S. Düsterer, E. Förster, C. Fortmann, S. H. Glenzer, S. Göde, G. Gregori, R. Irsig, T. Laarmann, H. J. Lee, B. Li, K. H. Meiwes-Broer, J. Mithen, B. Nagler, A. Przystawik, H. Redlin, R. Redmer, H. Reinholz, G. Röpke, F. Tavella, R. Thiele, J. Tiggesbäumker, S. Toleikis, I. Uschmann, S. M. Vinko, T. Whitcher, U. Zastrau. B. Ziaja, and T. Tschentscher, Phys. Rev. Lett., 104, 125002(2010).
[5] K. C. Prince, E. Allaria, C. Callegari, R. Cucini, G. De Ninno, S. Di Mitri, B. Diviacco, E. Ferrari, P. Finetti, D. Gauthier, L. Giannessi, N. Mahne, G. Penco, O. Plekan, L. Raimondi, P. Rebernik, E. Roussel, C. Svetina, M. Trovò, M. Zangrando, M. Negro, P. Carpeggiani, M. Reduzzi, G. Sansone, A. N. Grum-Grzhimailo, E. V. Gryzlova, S. I. Strakhova, K. Bartschat, N. Douguet, J. Venzke, D. Iablonskyi, Y. Kumagai, T. Takanashi, K. Ueda, A. Fischer, M. Coreno, F. Stienkemeier, Y. Ovcharenko, T. Mazza, M. Meyer. Nat. Photonics, 10, 176(2016).
[6] P. K. Maroju, C. Grazioli, M. Di Fraia, M. Moioli, D. Ertel, H. Ahmadi, O. Plekan, P. Finetti, E. Allaria, L. Giannessi, G. De Ninno, C. Spezzani, G. Penco, S. Spampinati, A. Demidovich, M. B. Danailov, R. Borghes, G. Kourousias, C. E. Sanches Dos Reis, F. Billé, A. A. Lutman, R. J. Squibb, R. Feifel, P. Carpeggiani, M. Reduzzi, T. Mazza, M. Meyer, S. Bengtsson, N. Ibrakovic, E. R. Simpson, J. Mauritsson, T. Csizmadia, M. Dumergue, S. Kühn, H. Nandiga Gopalakrishna, D. You, K. Ueda, M. Labeye, J. E. Bækhøj, K. J. Schafer, E. V. Gryzlova, A. N. Grum-Grzhimailo, K. C. Prince, C. Callegari, G. Sansone. Nature, 578, 386(2020).
[7] D. NölleProceedings of the 39th International Free Electron Laser Conference. , in (), p. ., 766(2019).
[8] B. Faatz, E. Plönjes, S. Ackermann, A. Agababyan, V. Asgekar, V. Ayvazyan, S. Baark, N. Baboi, V. Balandin, N. Bargen, Y. Bican, O. Bilani, J. Bödewadt, M. Böhnert, R. Böspflug, S. Bonfigt, H. Bolz, F. Borges, O. Borkenhagen, M. Brachmanski, M. Braune, A. Brinkmann, O. Brovko, T. Bruns, P. Castro, J. Chen, M. K. Czwalinna, H. Damker, W. Decking, M. Degenhardt, A. Delfs, T. Delfs, H. Deng, M. Dressel, H. T. Duhme, S. Düsterer, H. Eckoldt, A. Eislage, M. Felber, J. Feldhaus, P. Gessler, M. Gibau, N. Golubeva, T. Golz, J. Gonschior, A. Grebentsov, M. Grecki, C. Grün, S. Grunewald, K. Hacker, L. Hänisch, A. Hage, T. Hans, E. Hass, A. Hauberg, O. Hensler, M. Hesse, K. Heuck, A. Hidvegi, M. Holz, K. Honkavaara, H. Höppner, A. Ignatenko, J. Jäger, U. Jastrow, R. Kammering, S. Karstensen, A. Kaukher, H. Kay, B. Keil, K. Klose, V. Kocharyan, M. Köpke, M. Körfer, W. Kook, B. Krause, O. Krebs, S. Kreis, F. Krivan, J. Kuhlmann, M. Kuhlmann, G. Kube, T. Laarmann, C. Lechner, S. Lederer, A. Leuschner, D. Liebertz, J. Liebing, A. Liedtke, L. Lilje, T. Limberg, D. Lipka, B. Liu, B. Lorbeer, K. Ludwig, H. Mahn, G. Marinkovic, C. Martens, F. Marutzky, M. Maslocv, D. Meissner, N. Mildner, V. Miltchev, S. Molnar, D. Mross, F. Müller, R. Neumann, P. Neumann, D. Nölle, F. Obier, M. Pelzer, H. B. Peters, K. Petersen, A. Petrosyan, G. Petrosyan, L. Petrosyan, V. Petrosyan, A. Petrov, S. Pfeiffer, A. Piotrowski, Z. Pisarov, T. Plath, P. Pototzki, M. J. Prandolini, J. Prenting, G. Priebe, B. Racky, T. Ramm, K. Rehlich, R. Riedel, M. Roggli, M. Röhling, J. Rönsch-Schulenburg, J. Rossbach, V. Rybnikov, J. Schäfer, J. Schaffran, H. Schlarb, G. Schlesselmann, M. Schlösser, P. Schmid, C. Schmidt, F. Schmidt-Föhre, M. Schmitz, E. Schneidmiller, A. Schöps, M. Scholz, S. Schreiber, K. Schütt, U. Schütz, H. Schulte-Schrepping, M. Schulz, A. Shabunov, P. Smirnov, E. Sombrowski, A. Sorokin, B. Sparr, J. Spengler, M. Staack, M. Stadler, C. Stechmann, B. Steffen, N. Stojanovic, V. Sychev, E. Syresin, T. Tanikawa, F. Tavella, N. Tesch, K. Tiedtke, M. Tischer, R. Treusch, S. Tripathi, P. Vagin, P. Vetrov, S. Vilcins, M. Vogt, A. Z. Wagner, T. Wamsat, H. Weddig, G. Weichert, H. Weigelt, N. Wentowski, C. Wiebers, T. Wilksen, A. Willner, K. Wittenburg, T. Wohlenberg, J. Wortmann, W. Wurth, M. Yurkov, I. Zagorodnov, J. Zemella. New J. Phys., 18, 062002(2016).
[9] J. N. GalaydaProceedings of the 9th International Particle Accelerator Conference. , in (), p. ., 18(2018).
[10] Z. Zhu, Z. T. Zhao, D. Wang, Z. H. Yang, L. YinProceedings of the 38th International Free Electron Laser Conference. , , , , and , in (), p. ., 182(2017).
[11] W. Ackermann, G. Asova, V. Ayvazyan, A. Azima, N. Baboi, J. Bähr, V. Balandin, B. Beutner, A. Brandt, A. Bolzmann, R. Brinkmann, O. I. Brovko, M. Castellano, P. Castro, L. Catani, E. Chiadroni, S. Choroba, A. Cianchi, J. T. Costello, D. Cubaynes, J. Dardis, W. Decking, H. Delsim-Hashemi, A. Delserieys, G. Di Pirro, M. Dohlus, S. Düsterer, A. Eckhardt, H. T. Edwards, B. Faatz, J. Feldhaus, K. Flöttmann, J. Frisch, L. Fröhlich, T. Garvey, U. Gensch, C. Gerth, M. Görler, N. Golubeva, H. J. Grabosch, M. Grecki, O. Grimm, K. Hacker, U. Hahn, J. H. Han, K. Honkavaara, T. Hott, M. Hüning, Y. Ivanisenko, E. Jaeschke, W. Jalmuzna, T. Jezynski, R. Kammering, V. Katalev, K. Kavanagh, E. T. Kennedy, S. Khodyachykh, K. Klose, V. Kocharyan, M. Körfer, M. Kollewe, W. Koprek, S. Korepanov, D. Kostin, M. Krassilnikov, G. Kube, M. Kuhlmann, C. L. S. Lewis, L. Lilje, T. Limberg, D. Lipka, F. Löhl, H. Luna, M. Luong, M. Martins, M. Meyer, P. Michelato, V. Miltchev, W. D. Möller, L. Monaco, W. F. O. Müller, O. Napieralski, O. Napoly, P. Nicolosi, D. Nölle, T. Nuñez, A. Oppelt, C. Pagani, R. Paparella, N. Pchalek, J. Pedregosa-Gutierrez, B. Petersen, B. Petrosyan, G. Petrosyan, L. Petrosyan, J. Pflüger, E. Plönjes, L. Poletto, K. Pozniak, E. Prat, D. Proch, P. Pucyk, P. Radcliffe, H. Redlin, K. Rehlich, M. Richter, M. Roehrs, J. Roensch, R. Romaniuk, M. Ross, J. Rossbach, V. Rybnikov, M. Sachwitz, E. L. Saldin, W. Sandner, H. Schlarb, B. Schmidt, M. Schmitz, P. Schmüser, J. R. Schneider, E. A. Schneidmiller, S. Schnepp, S. Schreiber, M. Seidel, D. Sertore, A. V. Shabunov, C. Simon, S. Simrock, E. Sombrowski, A. A. Sorokin, P. Spanknebel, R. Spesyvtsev, L. Staykov, B. Steffen, F. Stephan, F. Stulle, H. Thom, K. Tiedtke, M. Tischer, S. Toleikis, R. Treusch, D. Trines, I. Tsakov, E. Vogel, T. Weiland, H. Weise, M. Wellhöfer, M. Wendt, I. Will, A. Winter, K. Wittenburg, W. Wurth, P. Yeates, M. V. Yurkov, I. Zagorodnov, K. Zapfe. Nat. Photonics, 1, 336(2007).
[12] P. Emma, R. Akre, J. Arthur, R. Bionta, C. Bostedt, J. Bozek, A. Brachmann, P. Bucksbaum, R. Coffee, F. J. Decker, Y. Ding, D. Dowell, S. Edstrom, A. Fisher, J. Frisch, S. Gilevich, J. Hastings, G. Hays, P. Hering, Z. Huang, R. Iverson, H. Loos, M. Messerschmidt, A. Miahnahri, S. Moeller, H. D. Nuhn, G. Pile, D. Ratner, J. Rzepiela, D. Schultz, T. Smith, P. Stefan, H. Tompkins, J. Turner, J. Welch, W. White, J. Wu, G. Yocky, J. Galayda. Nat. Photonics, 4, 641(2010).
[13] D. Ratner, R. Abela, J. Amann, C. Behrens, D. Bohler, G. Bouchard, C. Bostedt, M. Boyes, K. Chow, D. Cocco, F. J. Decker, Y. Ding, C. Eckman, P. Emma, D. Fairley, Y. Feng, C. Field, U. Flechsig, G. Gassner, J. Hastings, P. Heimann, Z. Huang, N. Kelez, J. Krzywinski, H. Loos, A. Lutman, A. Marinelli, G. Marcus, T. Maxwell, P. Montanez, S. Moeller, D. Morton, H. D. Nuhn, N. Rodes, W. Schlotter, S. Serkez, T. Stevens, J. Turner, D. Walz, J. Welch, J. Wu. Phys. Rev. Lett., 114, 054801(2015).
[14] D. Pile. Nat. Photonics, 5, 456(2011).
[15] L. H. Yu, M. Babzien, I. Ben-Zvi, L. F. DiMauro, A. Doyuran, W. Graves, E. Johnson, S. Krinsky, R. Malone, I. Pogorelsky, J. Skaritka, G. Rakowsky, L. Solomon, X. J. Wang, M. Woodle, V. Yakimenko, S. G. Biedron, J. N. Galayda, E. Gluskin, J. Jagger, V. Sajaev, I. Vasserman. Science, 289, 932(2000).
[16] B. Liu, W. B. Li, J. H. Chen, Z. H. Chen, H. X. Deng, J. G. Ding, Y. Fan, G. P. Fang, C. Feng, L. Feng, Q. Gu, M. Gu, C. Guo, D. Z. Huang, M. M. Huang, W. H. Huang, Q. K. Jia, T. H. Lan, Y. B. Leng, D. G. Li, W. M. Li, X. Li, G. Q. Lin, L. Shang, L. Shen, C. X. Tang, G. L. Wang, L. Wang, R. Wang, X. T. Wang, Z. S. Wang, Z. S. Wang, H. F. Yao, K. R. Ye, L. X. Yin, L. Y. Yu, J. Q. Zhang, M. Zhang, M. Zhang, T. Zhang, W. Y. Zhang, S. P. Zhong, Q. G. Zhou, D. Wang, Z. T. Zhao. Phys. Rev. ST Accel. Beams, 16, 020704(2013).
[17] E. Allaria, P. Cinquegrana, S. Cleva, D. Cocco, M. Cornacchia, P. Craievich, I. Cudin, G. D’Auria, M. Dal Forno, M. Danailov, R. De Monte, G. De Ninno, P. Delgiusto, A. Demidovich, S. Di Mitri, B. Diviacco, A. Fabris, R. Fabris, W. Fawley, M. Zangrando. Nat. Photonics, 6, 699(2012).
[18] D. Xiang, G. Stupakov. Phys. Rev. ST Accel. Beams, 12, 030702(2009).
[19] C. Feng, H. Deng, M. Zhang, X. Wang, S. Chen, T. Liu, K. Zhou, D. Gu, Z. Wang, Z. Jiang, X. Li, B. Wang, W. Zhang, T. Lan, L. Feng, B. Liu, Q. Gu, Y. Leng, L. Yin, D. Wang, Z. Zhao, G. Wang, D. Xiang. Phys. Rev. Accel. Beams, 22, 050703(2019).
[20] Z. T. Zhao, D. Wang, J. H. Chen, Z. H. Chen, H. X. Deng, J. G. Ding, C. Feng, Q. Gu, M. M. Huang, T. H. Lan, Y. B. Leng, D. G. Li, G. Q. Lin, B. Liu, E. Prat, X. T. Wang, Z. S. Wang, K. R. Ye, L. Y. Yu, H. O. Zhang, J. Q. Zhang, M. Zhang, M. Zhang, T. Zhang, S. P. Zhong, Q. G. Zhou. Nat. Photonics, 6, 360(2012).
[21] P. R. Ribič, A. Abrami, L. Badano, M. Bossi, H.-H. Braun, N. Bruchon, F. Capotondi, D. Castronovo, M. Cautero, P. Cinquegrana, M. Coreno, M. E. Couprie, I. Cudin, M. B. Danailov, G. De Ninno, A. Demidovich, S. Di Mitri, B. Diviacco, W. M. Fawley, C. Feng, M. Ferianis, E. Ferrari, L. Foglia, F. Frassetto, G. Gaio, D. Garzella, A. Ghaith, F. Giacuzzo, L. Giannessi, V. Grattoni, S. Grulja, E. Hemsing, F. Iazzourene, G. Kurdi, M. Lonza, N. Mahne, M. Malvestuto, M. Manfredda, C. Masciovecchio, P. Miotti, N. S. Mirian, I. P. Nikolov, G. M. Penco, G. Penn, L. Poletto, M. Pop, E. Prat, E. Principi, L. Raimondi, S. Reiche, E. Roussel, R. Sauro, C. Scafuri, P. Sigalotti, S. Spampinati, C. Spezzani, L. Sturari, M. Svandrlik, T. Tanikawa, M. Trovó, M. Veronese, D. Vivoda, D. Xiang, M. Zaccaria, D. Zangrando, M. Zangrando, E. M. Allaria. Nat. Photonics, 13, 555(2019).
[22] A. Wituschek, L. Bruder, E. Allaria, U. Bangert, M. Binz, R. Borghes, C. Callegari, G. Cerullo, P. Cinquegrana, L. Giannessi, M. Danailov, A. Demidovich, M. Di Fraia, M. Drabbels, R. Feifel, T. Laarmann, R. Michiels, N. S. Mirian, M. Mudrich, I. Nikolov, F. H. O’Shea, G. Penco, P. Piseri, O. Plekan, K. C. Prince, A. Przystawik, P. R. Ribič, G. Sansone, P. Sigalotti, S. Spampinati, C. Spezzani, R. J. Squibb, S. Stranges, D. Uhl, F. Stienkemeier. Nat. Commun., 11, 883(2020).
[23] G. Penco, G. Perosa, E. Allaria, S. Di Mitri, E. Ferrari, L. Giannessi, S. Spampinati, C. Spezzani, M. Veronese. Phys. Rev. Accel Beams, 23, 120704(2020).
[24] Q. K. Jia. Nucl. Instrum. Methods Phys. Res. A Accel. Spectrom. Detect. Assoc. Equip., 1015, 165767(2021).
[25] S. Ackermann, B. Faatz, V. Grattoni, M. M. Kazemi, T. Lang, C. Lechner, G. Paraskaki, J. Zemella, G. Geloni, S. Serkez, T. Tanikawa, W. Hillert. Phys. Rev. Accel. Beams, 23, 071302(2020).
[26] G. Paraskaki, V. Grattoni, T. Lang, J. Zemella, B. Faatz, W. Hillert. Phys. Rev. Accel. Beams, 24, 034801(2021).
[27] D. J. Dunning, N. R. Thompson, B. W. J. McNeil. J. Modern Opt., 58, 1362(2011).
[28] L. Zeng, W. L. Qin, G. Zhao, S. L. Huang, Y. T. Ding, Z. R. Huang, G. Marcus, K. X. Liu. Chin. Phys. C, 40, 098102(2016).
[29] X. Wang, C. Feng, B. Faatz, W. Zhang, Z. Zhao. , , , , and , ().(2021). arXiv:2103.11971
[30] M. Labat, M. Bellaveglia, M. Bougeard, B. Carré, F. Ciocci, E. Chiadroni, A. Cianchi, M. E. Couprie, L. Cultrera, M. Del Franco, G. Di Pirro, A. Drago, M. Ferrario, D. Filippetto, F. Frassetto, A. Gallo, D. Garzella, G. Gatti, L. Giannessi, G. Lambert, A. Mostacci, A. Petralia, V. Petrillo, L. Poletto, M. Quattromini, J. V. Rau, C. Ronsivalle, E. Sabia, M. Serluca, I. Spassovsky, V. Surrenti, C. Vaccarezza, C. Vicario. Phys. Rev. Lett., 107, 224801(2011).
[31] J. Yan, Z. Gao, Z. Qi, K. Zhang, K. Zhou, T. Liu, S. Chen, C. Feng, C. Li, L. Feng, T. Lan, W. Zhang, X. Wang, X. Li, Z. Jiang, B. Wang, Z. Wang, D. Gu, M. Zhang, H. Deng, Q. Gu, Y. Leng, L. Yin, B. Liu, D. Wang, Z. Zhao. Phys. Rev. Lett., 126, 084801(2021).
[32] J. W. Yan, N. S. Huang, H. X. Deng, B. Liu, D. Wang, Z. T. Zhao. Adv. Photonics, 3, 045003(2021).
[33] L. Giannessi, E. Allaria, L. Badano, D. Castronovo, P. Cinquegrana, P. Craievich, M. B. Danailov, G. D’Auria, A. Demidovitch, G. De Ninno, S. Di Mitri, B. Diviacco, W. M. Fawley, E. Ferrari, L. Froehlich, G. Gaio, R. Ivanov, E. Karantzoulis, B. Mahieu, N. Mahne, I. Nikolov, F. Parmigiani, G. Penco, L. Raimondi, C. Serpico, P. Sigalotti, S. Spampinati, C. Spezzani, M. Svandrlik, C. Svetina, M. Trovò, M. Veronese, D. Zangrando, M. ZangrandoProceedings of FEL2012. , , , , , , , , , , , , , , , , , , , , , , , , , , , , , , , , , , in (), p. ., 13(2012).
[34] K.-J. Kim, Z. Huang, R. Lindberg. Synchrotron Radiation and Free-Electron Lasers: Principles of Coherent X-Ray Generation(2017).
[35] E. Prat, M. Calvi, R. Ganter, S. Reiche, T. Schietinger, T. Schmidt. J. Synchrot. Radiat., 23, 861(2016).
[36] W. Qin, F. Curbis, J. Andersson, V. Goryashko, L. Isaksson, B. Kyle, F. Lindau, E. Mansten, M. Pop, P. Salen, H. Tarawneh, P. F. Tavares, S. Thorin, A. Vorozhtsov, S. Werin. J. Synchrot. Radiat., 28, 707(2021).
[37] S. Reiche. Nucl. Instrum. Methods Phys. Res. Sect. A Accel. Spectrom. Detect. Associat. Equip., 429, 243(1999).
[38] R. Wilcox, G. Marcus, G. Penn, T. Metzger, M. SchultzeProceedings of FEL2013. , , , and , in (), p. ., 697(2013).
[39] V. Balandin, N. GolubevaProceedings of 8th International Particle Accelerator Conference. and , in (), p. ., 686(2017).
[40] P. Craievich, S. Di Mitri, M. Milloch, G. Penco, F. Rossi. Phys. Rev. ST Accel. Beams, 16, 090401(2013).
[41] P. Craievich, S. D. Mitri, A. ZholentsProceedings of the 10th European Particle Accelerator Conference. , , and , in (), p. ., 2880(2006).
[42] G. Penco, M. Danailov, A. Demidovich, E. Allaria, G. De Ninno, S. Di Mitri, W. M. Fawley, E. Ferrari, L. Giannessi, M. Trovó. Phys. Rev. Lett., 112, 044801(2014).
[43] Z. Huang, D. Ratner, G. Stupakov, D. XiangProceedings of FEL2009. , , . and , in (), p. ., 127(2009).
[44] K. Zhang, Z. Qi, C. Feng, H. Deng, D. Wang, Z. Zhao. Nucl. Instrum. Methods Phys. Res. Sect. A Accel. Spectrom. Detect. Assoc. Equip., 854, 3(2017).
[45] N. Kroll, P. Morton, M. Rosenbluth. IEEE J. Quantum. Elect., 17, 1436(1981).
[46] E. A. Schneidmiller, M. V. Yurkov. Phys. Rev. ST Accel. Beams, 18, 030705(2015).
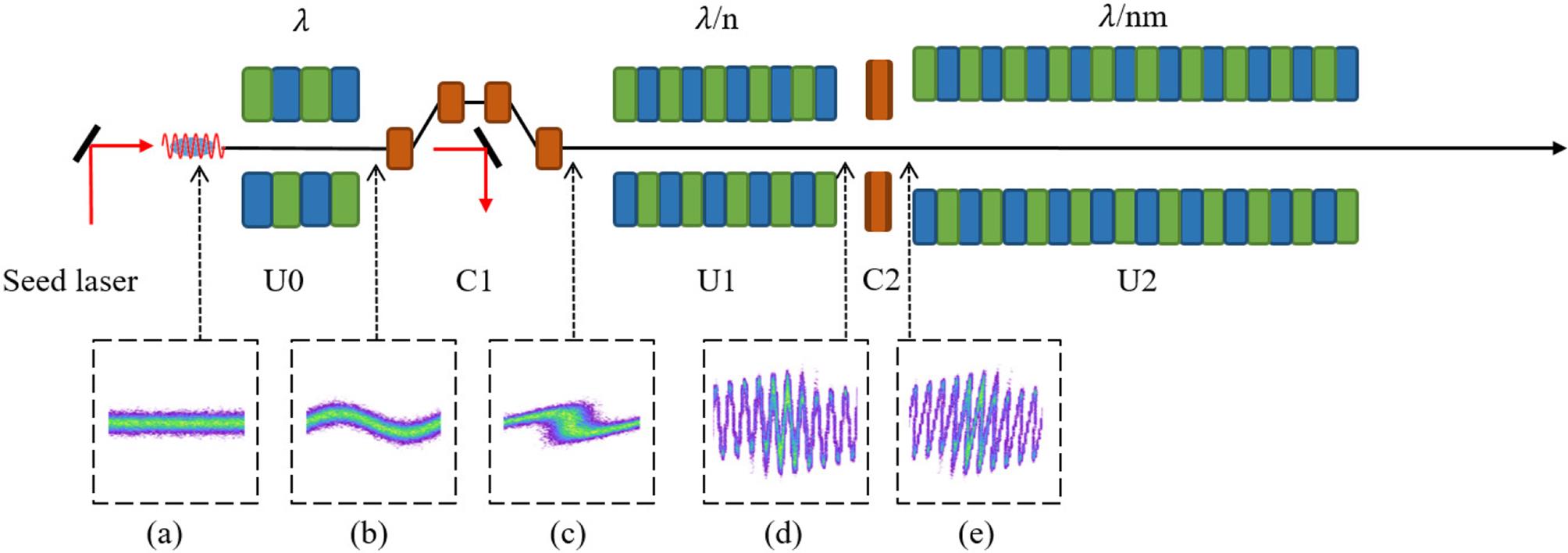
Set citation alerts for the article
Please enter your email address