
- Photonics Research
- Vol. 10, Issue 6, 1380 (2022)
Abstract
1. INTRODUCTION
Conventional optical elements achieve specific optical functions based on the gradual phase changes accumulated along the propagation path, leading to a large form factor that is not compatible with the miniaturized, lightweight, and compact systems [1,2]. Therefore, planar optics components have received extensive attention in recent years benefiting from the compact and ultrathin design and excellent manipulation capability in multi-dimensional physical parameters [3]. The generalized laws of reflection and refraction offer the theoretical explanation for the principle of unique performance in planar optics components, leading to arbitrary wavefront modulation due to the phase discontinuities in light propagation [4]. In general, the phase discontinuities can be implemented by geometric phase, dynamic phase, and so forth. Compared with the dynamic phase arising from the optical path difference in propagation, the geometric phase, also known as Pancharatnam–Berry (PB) phase, originates from the photonic spin–orbit interaction in asymmetric anisotropic structures [5,6]. In contrast with the dynamic phase that is adjusted by the equivalent refractive index of the material, the PB phase is a broadband non-dispersion phase modulation method that is only related to the rotation angle of the anisotropic structure [7]. Therefore, PB phase has been widely used in multitudinous practical applications due to its precise phase control ability and robustness against fabrication tolerances.
Past decades have witnessed extensive efforts on geometric phase. Among these applications, metasurfaces are the most representative planar optical components with promising potential and application value. Metasurfaces, the equivalent artificial two-dimensional (2D) metamaterials, generally comprise plentiful sub-wavelength nanostructures arranged in accordance with specific orders, which can be applied to accurately control multiple physical characteristics of the light field, including polarization, amplitude, and phase [8,9]. Geometric phase metasurfaces have been widely used in optical lenses [10,11], catenary optics [12,13], the spin Hall effect [14], holograms [15,16], vortex beam generators [17,18], and so on. Recently, generalized geometric phase in rotationally symmetric meta-atoms has been demonstrated to enrich the understanding of the geometric phase as well as light–matter interaction in nanophotonics [19]. However, although metasurfaces obtain precise and flexible control capabilities at sub-wavelength scales, the preparation of the metasurfaces comprising plentiful nanopillars or nanoholes is facing huge challenges especially in large-area manufacturing [20]. Currently, numerous nanopatterning techniques have been extensively applied in the nanopatterning, such as focused ion-beam (FIB) milling, electron-beam lithography (EBL), ion-beam lithography (IBL), and atomic layer deposition (ALD). These techniques suffer from high cost and low throughput and are not compatible with the low-cost, high-efficiency, and large-area demands in mass nanofabrication [21].
Actually, the anisotropic liquid crystals (LCs) can also realize a series of impressive functions by utilizing the designed method in geometric phase metasurfaces. To a certain extent, LCs can achieve the arbitrary wavefront manipulation by introducing discontinuous phase gradients at the interface between the two media, which is consistent with the generalized laws of reflection and refraction; thus, the planar LC optical elements can be considered as a kind of generalized metasurface. Here we define the proposed device as a polymer LC metasurface, which is the same as the previous report [22]. On the one hand, although optical lithography has attracted a great deal of interest and shown promising applications in low-cost and large-area nanofabrication, the expensive laser setups and complex processing inevitably restrict their applications. The gradual maturity of LC production lines provides the low-cost and large-scale production for LC metasurfaces, which can be adopted to offer a cost-effective and high-throughput method for the fabrication of metasurfaces. On the other hand, compared to conventional diffraction optical elements, LCs also revealed unparalleled superiority in terms of the work efficiency, dynamic tunability, and processing difficulty. So far, LCs have been widely applied in displays, optical imaging, holography, and so forth. In particular, LC displays and spatial light modulators are the most representative and widespread applications in modern engineering and technology. Recently, geometric phase LCs based on light alignment technology have received tremendous attention and impressive progress in multifarious application scenarios, including near-eye display [23], virtual reality/augmented reality (VR/AR) display [24], integral imaging [25], spiral phase modulation [26,27], beam splitting [28,29], polarization gratings [30], telescope [31], and so on. It is worth mentioning that one of the most widely used applications is LC metalenses, which have received extensive attention due to the planar design with the extreme optical performance and low-cost manufacturing. The planar high-efficiency LC metalenses also have great superiority in advanced optical systems due to the high integration and light weight requirements. Therefore, LC metalenses are of great significance and of great potential in advanced optical imaging systems.
As one of the most representative applications in planar optical elements, polarization-multiplexing metasurfaces have received sufficient attention and research to achieve the multitudinous functions, including lenses [32,33] and holography [34], while these polarization-multiplexing devices cannot balance bandwidth, efficiency, and multifunctionality efficiently. Among these applications, the polarization-multiplexing lenses based on PB phase obtain high error tolerance in nanofabrication, and the ultrabroadband characteristics can greatly broaden the working bandwidth of the designed lenses. In addition, polymerized LC metalenses, as a representative planar optical component based on PB phase, achieve nearly 100% polarization conversion efficiency once the thickness of the LC layer can satisfy the half-wave condition. Previously reported LCs were devoted to the multi-foci functions such as a PB-phase-based polarization-multiplexing bifocal lenses [35] or LC integrated metalenses [36], while the different polarization-switchable focusing behaviors are rarely realized, which greatly limits the application scenarios of the proposed polarization-multiplexing LC lenses.
In this paper, broadband high-efficiency polymerized liquid crystal metasurfaces (BHPLCMs) are proposed for polarization-switchable focusing functions in the visible based on photonic spin–orbit interaction. Our proposed method can achieve excellent focusing performance simultaneously under LCP and RCP incident light based on wavefront engineering and holographic synthesis within the working bandwidth, leading to the enormous progress in the practical application scenarios. The experimental results are consistent with the simulated results, which can indicate that the spin-multiplexed method can be implemented to achieve the polarization-switchable functions. The proposed BHPLCMs are expected to have promising applications in microscopy, VR/AR, and optical interconnections.
2. DESIGN AND METHODS
The designed BHPLCMs are composed of plentiful anisotropic LC molecules with different rotation directions deposed on a glass substrate. In this work, two different BHPLCMs are designed to achieve different focusing functions: one can realize the diffraction-limited focusing to sub-diffraction focusing with 0.8 times Abbe diffraction limit, and the other can achieve the diffraction-limited focusing to the focusing vortex beam. Therefore, the planar polarization-multiplexing LC-polymer metasurfaces are expected to have flexible imaging functions based on the polarization-multiplexing method. As shown in Fig. 1, the proposed BHPLCMs can achieve different focusing modes under the different incident light with the left-circularly polarized (LCP) and right-circularly polarized (RCP) states.
Figure 1.Schematic diagram of broadband high-efficiency polymerized liquid crystal metasurfaces. The first row indicates that the designed
A. Design of the Polymerized LC Metasurfaces
Anisotropic materials, as an important characteristic of LCs, are an indispensable approach to control the polarization information of the incident light effectively. Theoretically, the design of PB-phase-based LC metasurfaces can be summarized as deriving the corresponding rotation angle to achieve the ideal geometric phase modulation of LC molecules through the Jones matrix, which establishes the relationship between the orientation angle (
Hence, the required ideal phase wavefront can be designed based on the geometric
B. Design of the BHPLCMs
Based on the above design scheme, polarization-multiplexing LC metalenses are proposed to further improve the flexibility in the actual application scenarios, and the key to realizing such polarization-multiplexing LC metasurfaces mainly lies in the capability of a single optical element to simultaneously achieve the different function under different incident light. Theoretically, the required phase distribution for the polarization-multiplexing LC lens is governed by
In this work, we designed two BHPLCMs with different focusing modes; for
The binary super-oscillation phase
For
Here, the designed wavelength
3. EXPERIMENT AND DISCUSSION
A. Fabrication of the BHPLCMs
After clarifying the required wavefront phase of the proposed BHPLCMs, the rotation angle of the LC molecules at the corresponding position can be determined according to the geometric phase theory. Subsequently, the required phase profile is discretized into 18-order phase (i.e., 0,
Figure 2.Fabrication and characterization of proposed BHPLCMs. (a) Schematic diagram of the fabrication procedure of BHPLCMs. (b) POM images of
B. Optical Performance of BHPLCMs
To further characterize the optical performance of our proposed BHPLCMs, the theoretical simulation results and experimental results are calculated to verify the validity of the polarization-multiplexing method. As shown in Fig. 3, a self-built optical equipment after beam expansion and collimation is utilized to detect the corresponding field distributions in the operating bandwidth. The system, illuminated by a supercontinuum laser source (NKT SuperK Extreme 15) equipped with a tunable filter (NKT Photonics, SuperK SELECT), is through polarizer 1 to achieve the polarization of the incident light. Certainly, polarizer 1 can be regarded as an attenuator to choose a suitable incident light field intensity. The collimator and beam expander are employed to ensure the uniform parallel incident beam on the proposed BHPLCMs. Hence, polarizer 2 and quarter-wave plate 1 (QWP1) are used to yield the LCP and RCP incidence. The QWP2 and polarizer 3 are selected to filter the co-polarization part, although this part is negligible for the final focusing performance benefited from the high PCR of LC metalenses, while it is necessary for the accuracy of efficiency measurement. Finally, the incident light (LCP/RCP) on the BHPLCMs will be focused and collected on the CCD (Daheng MER-310-12UC) mounted on a motorized stage, which is applied to measure light field distributions along the propagation direction.
Figure 3.Schematic of the experimental setup. The dashed box, physical map of the
First of all, the
The corresponding focal lengths for incident light of 480 nm, 530 nm, 580 nm, 633 nm, and 670 nm can be deduced from Eq. (12) as 593 mm, 537 mm, 491 mm, 450 mm, and 425 mm, respectively. The different focal plane positions at different incident wavelengths are switched by controlling the position of the CCD placed on the electric stage, and the switching focusing function at the single wavelength can be realized by rotating the QWP 90°. The focusing performance located at the left and center of Fig. 4 indicated the broadband characteristics of
Figure 4.Simulated and experimental light distributions at the focal plane for
Simultaneously, such a super-oscillatory wavefront is accompanied with a needle-like light field with a long focal depth, which is crucial for improving the robustness in practical applications. Accordingly, the axial light intensity distributions of the
Figure 5.Simulated and experimental light distributions along the propagation direction for
To further prove the universality of the proposed method, we designed
Figure 6.Simulated and experimental light distributions at the focal plane for
Subsequently, we also paid attention to the light field distribution along the propagation direction under the incidence of LCP or RCP. Here, the axial light field distributions are simulated and measured around
Figure 7.Simulated and experimental light distributions along the propagation direction for
One of the most preeminent characteristics of our proposed BHPLCMs is the LC materials with high PCR. Therefore, a powermeter is adopted to measure the PCR and focusing efficiency of BHPLCMs at several discrete wavelengths accurately. Here, the co-polarization part of the light field should be filtered after passing the BHPLCMs, so we add QWP2 and polarizer 3 behind the LC lenses based on the self-built system as shown in Fig. 3 to maximize the accuracy of the measurement for PCR although the intensity of the cross-polarization part is negligible. After the detailed measurement, the PCRs of
Figure 8.Calculated FWHM, PCR, and focusing efficiency of simulated and experimental results for
Obviously, the proposed
Indeed, the super-resolution ability can be further enhanced by adding appropriate constraints for
4. CONCLUSION
In this work, broadband high-efficiency polarization-multiplexing LC metalenses are proposed and experimentally verified based on the spin-dependent phase conjugation. It can be found that the efficient polarization-switchable focusing function can be achieved by arranging LC molecules rationally with different rotation directions. The proposed
APPENDIX A: THE OPTIMIZATION OF BINARY SUPER-OSCILLATORY PHASE
In this work, the light intensity distribution at the focal plane position can be approximately calculated by Eq. (
APPENDIX B: THE INFLUENCE OF THE DIFFERENT DISCRETE PRECISION
Here, we calculate the different optical performances of
Figure 9.Optical performance under different discrete precision.
APPENDIX C: THE ANALYSIS FOR THE DIFFERENCE BETWEEN THE SIMULATED AND EXPERIMENTAL RESULTS
Here, taking
Figure 10.Influence of inhomogeneity of the incident light.
Figure 11.Influence of the fabrication errors.
APPENDIX D: THE ANALYSIS OF SUPER-RESOLUTION ABILITY
Theoretically, the super oscillation phenomenon can produce arbitrarily small focal spots, while the energy of the sub-diffraction focal spot decreases significantly accompanied by the high-intensity sidelobes. Therefore, it is difficult to achieve many excellent characteristics at the same time including FOV,
Design Parameters for the Super-Oscillatory Lenses with Different Super-Resolution Abilities
Serial Number | 1 | 2 | 3 |
---|---|---|---|
0.7 | 0.6 | 0.5 | |
∞ | 1.6 | 1 | |
0.1 | 0.1 | 0.1 | |
0.122, 0.224, 0.358, 0.47, 0.612, 0.774 | 0.298, 0.594, 0.85 | 0.440, 0.814 |
Hence, the optical performances of super-oscillatory phases with different super-resolution capabilities are simulated by the VAS theory as shown in Fig.
Figure 12.Contrast between the super-oscillatory spots with different super-resolution capabilities.
Figure 13.Optical performance of the
References
[1] N. Yu, F. Capasso. Flat optics with designer metasurfaces. Nat. Mater., 13, 139-150(2014).
[2] Y. Zhou, H. Zheng, I. I. Kravchenko, J. Valentine. Flat optics for image differentiation. Nat. Photonics, 14, 316-323(2020).
[3] X. Luo. Engineering optics 2.0: a revolution in optical materials, devices, and systems. ACS Photon., 5, 4724-4738(2018).
[4] N. Yu, P. Genevet, M. A. Kats, F. Aieta, J.-P. Tetienne, F. Capasso, Z. Gaburro. Light propagation with phase discontinuities: generalized laws of reflection and refraction. Science, 334, 333-337(2011).
[5] S. Pancharatnam. Generalized theory of interference and its applications. Proc. Indian Acad. Sci. A, 44, 398-417(1956).
[6] M. V. Berry. The adiabatic phase and Pancharatnam’s phase for polarized light. J. Mod. Opt., 34, 1401-1407(1987).
[7] D. Tang, C. Wang, Z. Zhao, Y. Wang, M. Pu, X. Li, P. Gao, X. Luo. Ultrabroadband superoscillatory lens composed by plasmonic metasurfaces for subdiffraction light focusing. Laser Photon. Rev., 9, 713-719(2015).
[8] X. Luo. Principles of electromagnetic waves in metasurfaces. Sci. China Phys. Mech. Astron., 58, 594201(2015).
[9] Z. Yue, J. Li, J. Li, C. Zheng, J. Liu, G. Wang, H. Xu, M. Chen, Y. Zhang, Y. Zhang. Terahertz metasurface zone plates with arbitrary polarizations to a fixed polarization conversion. Opto-Electron. Sci., 1, 210014(2022).
[10] M. Khorasaninejad, W. T. Chen, R. C. Devlin, J. Oh, A. Y. Zhu, F. Capasso. Metalenses at visible wavelengths: diffraction-limited focusing and subwavelength resolution imaging. Science, 352, 1190-1194(2016).
[11] Y. Wang, Q. Fan, T. Xu. Design of high efficiency achromatic metalens with large operation bandwidth using bilayer architecture. Opto-Electron. Adv., 4, 200008(2021).
[12] M. Pu, X. Li, X. Ma, Y. Wang, Z. Zhao, C. Wang, C. Hu, P. Gao, C. Huang, H. Ren. Catenary optics for achromatic generation of perfect optical angular momentum. Sci. Adv., 1, e1500396(2015).
[13] F. Zhang, M. Pu, X. Li, X. Ma, Y. Guo, P. Gao, H. Yu, M. Gu, X. Luo. Extreme-angle silicon infrared optics enabled by streamlined surfaces. Adv. Mater., 33, 2008157(2021).
[14] X. Luo, M. Pu, X. Li, X. Ma. Broadband spin Hall effect of light in single nanoapertures. Light Sci. Appl., 6, e16276(2017).
[15] Z. L. Deng, M. Jin, X. Ye, S. Wang, T. Shi, J. Deng, N. Mao, Y. Cao, B. O. Guan, A. Alù. Full-color complex-amplitude vectorial holograms based on multi-freedom metasurfaces. Adv. Funct. Mater., 30, 1910610(2020).
[16] H. Gao, X. Fan, W. Xiong, M. Hong. Recent advances in optical dynamic meta-holography. Opto-Electron. Adv., 4, 210030(2021).
[17] S. Zhang, P. Huo, W. Zhu, C. Zhang, P. Chen, M. Liu, L. Chen, H. J. Lezec, A. Agrawal, Y. Lu. Broadband detection of multiple spin and orbital angular momenta via dielectric metasurface. Laser Photon. Rev., 14, 2000062(2020).
[18] H. Gao, Y. Li, L. Chen, J. Jin, M. Pu, X. Li, P. Gao, C. Wang, X. Luo, M. Hong. Quasi-Talbot effect of orbital angular momentum beams for generation of optical vortex arrays by multiplexing metasurface design. Nanoscale, 10, 666-671(2018).
[19] X. Xie, M. Pu, J. Jin, M. Xu, Y. Guo, X. Li, P. Gao, X. Ma, X. Luo. Generalized Pancharatnam-Berry phase in rotationally symmetric meta-atoms. Phys. Rev. Lett., 126, 183902(2021).
[20] M. K. Chen, Y. Wu, L. Feng, Q. Fan, M. Lu, T. Xu, D. P. Tsai. Principles, functions, and applications of optical meta-lens. Adv. Opt. Mater., 9, 2001414(2021).
[21] X. Luo. Extraordinary Young’s interferences and super-diffraction laser lithography. Handbook of Laser Micro- and Nano-Engineering, 1-40(2020).
[22] Q. Xu, T. Sun, C. Wang. Coded liquid crystal metasurface for achromatic imaging in the broadband wavelength range. ACS Appl. Nano Mater., 4, 5068-5075(2021).
[23] T. Zhan, Y. H. Lee, G. Tan, J. Xiong, K. Yin, F. Gou, J. Zou, N. Zhang, D. Zhao, J. Yang. Pancharatnam–Berry optical elements for head-up and near-eye displays. J. Opt. Soc. Am. B, 36, D52-D65(2019).
[24] H. Chen, Y. Weng, D. Xu, N. V. Tabiryan, S.-T. Wu. Beam steering for virtual/augmented reality displays with a cycloidal diffractive waveplate. Opt. Express, 24, 7287-7298(2016).
[25] X. Shen, Y.-J. Wang, H.-S. Chen, X. Xiao, Y.-H. Lin, B. Javidi. Extended depth-of-focus 3D micro integral imaging display using a bifocal liquid crystal lens. Opt. Lett., 40, 538-541(2015).
[26] B. Wei, S. Liu, P. Chen, S. Qi, Y. Zhang, W. Hu, Y. Lu, J. Zhao. Vortex Airy beams directly generated via liquid crystal q-Airy-plates. Appl. Phys. Lett., 112, 121101(2018).
[27] W. Duan, P. Chen, S.-J. Ge, B.-Y. Wei, W. Hu, Y.-Q. Lu. Helicity-dependent forked vortex lens based on photo-patterned liquid crystals. Opt. Express, 25, 14059-14064(2017).
[28] M. W. Kudenov, M. Miskiewicz, N. Sanders, M. J. Escuti. Achromatic Wollaston prism beam splitter using polarization gratings. Opt. Lett., 41, 4461-4463(2016).
[29] K. Gao, C. McGinty, H. Payson, S. Berry, J. Vornehm, V. Finnemeyer, B. Roberts, P. Bos. High-efficiency large-angle Pancharatnam phase deflector based on dual-twist design. Opt. Express, 25, 6283-6293(2017).
[30] S. R. Nersisyan, N. V. Tabiryan, L. Hoke, D. M. Steeves, B. Kimball. Polarization insensitive imaging through polarization gratings. Opt. Express, 17, 1817-1830(2009).
[31] Z. He, K. Yin, S.-T. Wu. Miniature planar telescopes for efficient, wide-angle, high-precision beam steering. Light Sci. Appl., 10, 1(2021).
[32] K. Ou, F. Yu, G. Li, W. Wang, A. E. Miroshnichenko, L. Huang, P. Wang, T. Li, Z. Li, X. Chen. Mid-infrared polarization-controlled broadband achromatic metadevice. Sci. Adv., 6, eabc0711(2020).
[33] C. Yan, X. Li, M. Pu, X. Ma, F. Zhang, P. Gao, K. Liu, X. Luo. Midinfrared real-time polarization imaging with all-dielectric metasurfaces. Appl. Phys. Lett., 114, 161904(2019).
[34] F. Zhang, M. Pu, X. Li, P. Gao, X. Ma, J. Luo, H. Yu, X. Luo. All-dielectric metasurfaces for simultaneous giant circular asymmetric transmission and wavefront shaping based on asymmetric photonic spin–orbit interactions. Adv. Funct. Mater., 27, 1704295(2017).
[35] Y. Zhou, Y. Yuan, T. Zeng, X. Wang, D. Tang, F. Fan, S. Wen. Liquid crystal bifocal lens with adjustable intensities through polarization controls. Opt. Lett., 45, 5716-5719(2020).
[36] S. Zhou, Z. Shen, X. Li, S. Ge, Y. Lu, W. Hu. Liquid crystal integrated metalens with dynamic focusing property. Opt. Lett., 45, 4324-4327(2020).
[37] P. Yeh, C. Gu. Optics of Liquid Crystal Displays(1999).
[38] J. Kim, Y. Li, M. N. Miskiewicz, C. Oh, M. W. Kudenov, M. J. Escuti. Fabrication of ideal geometric-phase holograms with arbitrary wavefronts. Optica, 2, 958-964(2015).
[39] M. Berry, S. Popescu. Evolution of quantum superoscillations and optical superresolution without evanescent waves. J. Phys. A, 39, 6965(2006).
[40] X. Luo. Meta-surface-waves in digital optics. J. Phys. Photon., 2, 041003(2020).
[41] F. Qin, K. Huang, J. Wu, J. Teng, C. W. Qiu, M. Hong. A supercritical lens optical label-free microscopy: sub-diffraction resolution and ultra-long working distance. Adv. Mater., 29, 1602721(2017).
[42] F. Qin, B. Liu, L. Zhu, J. Lei, W. Fang, D. Hu, Y. Zhu, W. Ma, B. Wang, T. Shi. π-phase modulated monolayer supercritical lens. Nat. Commun., 12, 1(2021).
[43] C. Wang, D. Tang, Y. Wang, Z. Zhao, J. Wang, M. Pu, Y. Zhang, W. Yan, P. Gao, X. Luo. Super-resolution optical telescopes with local light diffraction shrinkage. Sci. Rep., 5, 18485(2015).
[44] X. Lu, Y. Guo, M. Pu, Y. Zhang, Z. Li, X. Li, X. Ma, X. Luo. Broadband achromatic metasurfaces for sub-diffraction focusing in the visible. Opt. Express, 29, 5947-5958(2021).
[45] E. T. Rogers, J. Lindberg, T. Roy, S. Savo, J. E. Chad, M. R. Dennis, N. I. Zheludev. A super-oscillatory lens optical microscope for subwavelength imaging. Nat. Mater., 11, 432-435(2012).
[46] D. Tang, J. Liu, L. Chen, J. Liu, X. Zhang. Reflective plasmonic super-oscillatory metasurfaces with simultaneous phase and amplitude controls for sub-diffraction focusing. J. Phys. D, 53, 154001(2020).
[47] S. Wang, P. C. Wu, V.-C. Su, Y.-C. Lai, C. H. Chu, J.-W. Chen, S.-H. Lu, J. Chen, B. Xu, C.-H. Kuan. Broadband achromatic optical metasurface devices. Nat. Commun., 8, 1(2017).
[48] W. T. Chen, A. Y. Zhu, V. Sanjeev, M. Khorasaninejad, Z. Shi, E. Lee, F. Capasso. A broadband achromatic metalens for focusing and imaging in the visible. Nat. Nanotechnol., 13, 220-226(2018).
[49] T. Liu, J. Tan, J. Liu, H. Wang. Vectorial design of super-oscillatory lens. Opt. Express, 21, 15090-15101(2013).
[50] Z. Li, T. Zhang, Y. Wang, W. Kong, J. Zhang, Y. Huang, C. Wang, X. Li, M. Pu, X. Luo. Achromatic broadband super-resolution imaging by super-oscillatory metasurface. Laser Photon. Rev., 12, 1800064(2018).
[51] X. Dai, F. Dong, K. Zhang, D. Liao, S. Li, Z. Shang, Y. Zhou, G. Liang, Z. Zhang, Z. Wen. Holographic super-resolution metalens for achromatic sub-wavelength focusing. ACS Photon., 8, 2294-2303(2021).
[52] G. H. Yuan, E. T. Rogers, N. I. Zheludev. Achromatic super-oscillatory lenses with sub-wavelength focusing. Light Sci. Appl., 6, e17036(2017).
[53] D. Tang, L. Chen, J. Liu. Visible achromatic super-oscillatory metasurfaces for sub-diffraction focusing. Opt. Express, 27, 12308-12316(2019).
[54] S. Wang, P. C. Wu, V.-C. Su, Y. C. Lai, M.-K. Chen, H. Y. Kuo, B. H. Chen, Y. H. Chen, T. T. Huang, J.-H. Wang. A broadband achromatic metalens in the visible. Nat. Nanotechnol., 13, 227-232(2018).
[55] X. Lu, Y. Guo, M. Pu, M. Xu, J. Jin, Z. Li, X. Li, X. Ma, X. Luo. Switchable polarization-multiplexed super-oscillatory metasurfaces for achromatic sub-diffraction focusing. Opt. Express, 28, 39024-39037(2020).
[56] P. Huo, C. Zhang, W. Zhu, M. Liu, S. Zhang, S. Zhang, L. Chen, H. J. Lezec, A. Agrawal, Y. Lu. Photonic spin-multiplexing metasurface for switchable spiral phase contrast imaging. Nano Lett., 20, 2791-2798(2020).
[57] A. Nemati, Q. Wang, M. Hong, J. Teng. Tunable and reconfigurable metasurfaces and metadevices. Opto-Electron. Adv., 1, 18000901(2018).
[58] T. Cao, M. Lian, X. Chen, L. Mao, K. Liu, J. Jia, Y. Su, H. Ren, S. Zhang, Y. Xu. Multi-cycle reconfigurable THz extraordinary optical transmission using chalcogenide metamaterials. Opto-Electron. Sci., 1, 210010(2022).
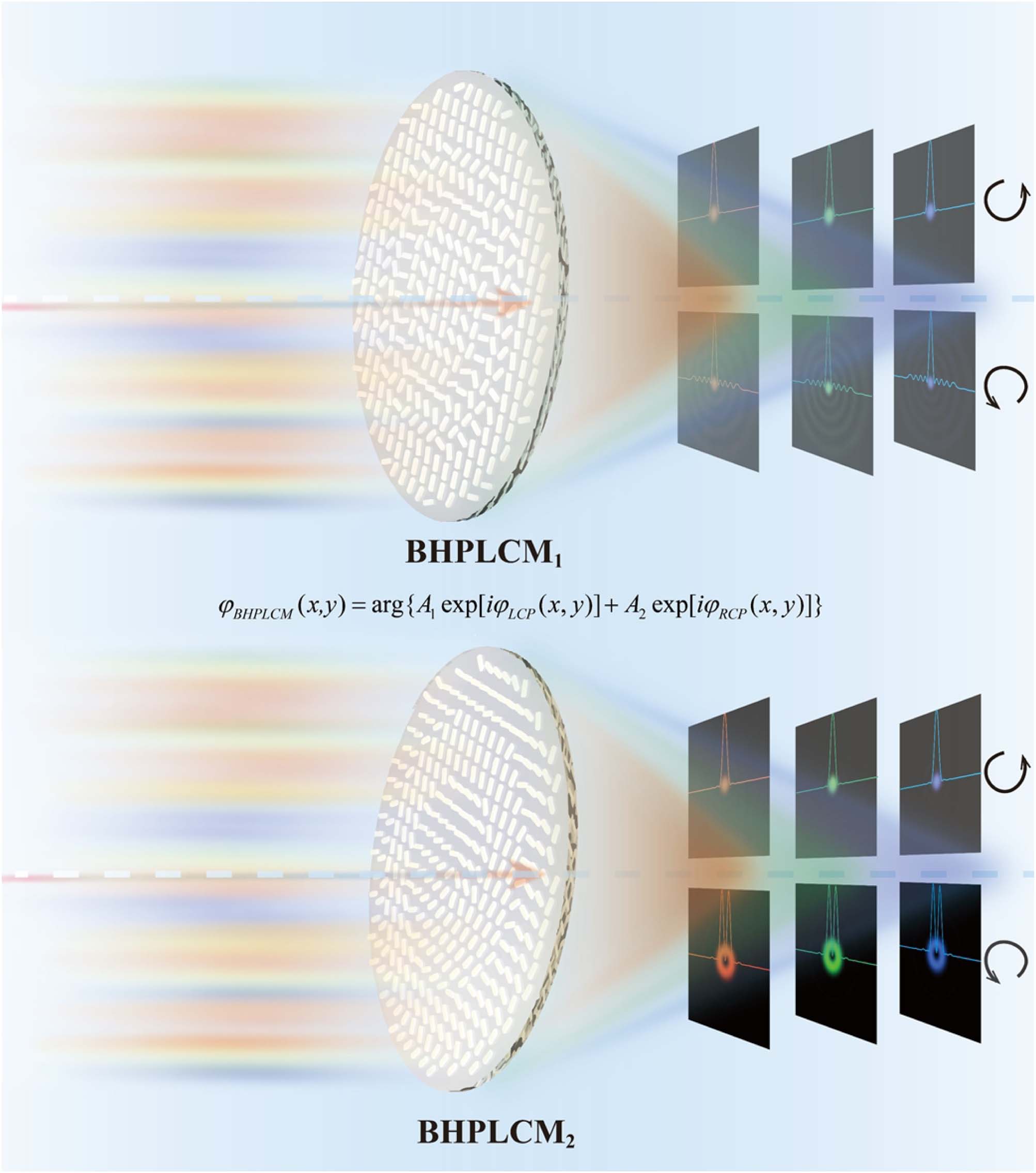
Set citation alerts for the article
Please enter your email address