
- Chinese Optics Letters
- Vol. 17, Issue 9, 090603 (2019)
Abstract
1. INTRODUCTION
In the early 17th century, German astronomer Kepler hypothesized that comet tails point away from the sun due to the force of solar radiation. Moreover, Maxwell’s electromagnetic theories also explain the light itself can produce radiation pressure. The development of optical manipulation technology began with the emergence of the laser beam in the 1960s. Ashikin, as a pioneer in 1970[
It is well-established that a cell is the basic unit of life. The study of cells can lead to a better understanding of the differences between living organisms as well as a better understanding of ourselves. Since Ashkin successfully trapped and manipulated the yeast cells by optical trapping[
A typical optical tweezers system consists of four parts, including the optical trapping device, mechanical measurement device, image capture device, and optical potential well displacement operation device[
Sign up for Chinese Optics Letters TOC. Get the latest issue of Chinese Optics Letters delivered right to you!Sign up now
Optical manipulation techniques can use a single light beam, a dual light beam, and even a multilight beam. Near the focus of the light beam, the forces have an effect on the particle. These forces can be divided into two types: (1) the scattering force along the propagation direction of the light beam to push the particle away, and (2) the gradient force along the gradient of the light field to pull the particle to the strongest position of the light field gradient. The basic principle of the optical trap is that the gradient force is larger than the scattering force, so the particle can be captured closed to the center of the focus spot. As Fig.
Figure 1.Stable 3D optical trapping near the focus area, where the gradient force dominates the micro-particle[
Generally, the theoretical models mainly depend on the size of the microparticles being trapped. The models can be divided into three cases. When the diameter
Optical tweezers have been developed over thirty years generating various types, such as novel-beam optical tweezers, near-field optical tweezers, hologram tweezers, plasmonic optical tweezers, and fiber optical tweezers[
2. FIBER OPTICAL TWEEZERS
2.1. Why Fiber Optical Tweezers?
We can divide optical tweezers into two types: traditional optical tweezers and fiber optical tweezers. Traditional optical tweezers often include several necessary optical elements such as an objective lens with high NA, a beam expander, a dichroic mirror, a light source, and reflective mirrors. The cost of traditional optical tweezers is high. Fiber optical tweezers usually include a light source and an optical fiber; the microscope only works as an observation device. Thus, fiber optical tweezers are usually simpler and cheaper than traditional tweezers.
One can find the differences between traditional optical tweezers and fiber optical tweezers from the comparison shown in Table
Spatial Optical Tweezers | Fiber Optical Tweezers |
---|---|
Complex structure Large size Large trapping force Limited operating area Unsuitable for trapping Multitrapping and complicated manipulation by using SLM Expensive Various optical fields | Simple structure Compact size Small trapping force Trapped particle moving with optical fiber High flexibility Suitable for trapping Simple multitrapping and low operability for multiparticles Low cost Limited optical field |
Single-particle trapping and manipulation |
Table 1. Comparison of Spatial Optical Tweezers and Optical Fiber Tweezers
Traditional optical tweezers are powerful tools for particle manipulation, especially when they are combined with a spatial light modulator (SLM). In the latter case, multitrapping and complicated manipulation can be realized. Although optical fiber tweezers are insufficient for manipulating multiparticles at will, they can trap several particles and arrange them simultaneously[
Traditional optical tweezers show much promise in living cell manipulation; however, they are bulky, and the fixed light path makes them unsuitable for cell trapping
2.2. Fabrication of Optical Fiber Tweezers
The key goal of optical tweezers is to create a large divergence angle to form enough optical gradient force. However, the output optical field from the unprocessed fiber tip usually has a narrow divergence angle, which cannot generate a powerful trapping force. Thus, understanding how to construct a focusing optical field with large divergence angle at the fiber tip is important. There are two key elements: optical fiber type and fiber tip structure.
Table
Type of Fabrication | Advantages | Disadvantages | Representative Works | Reference |
---|---|---|---|---|
Polishing/grinding | Easy to implement, low cost, reproducibility, no contact | Low focusing power, time-consuming (min–hours) | [ | |
Fusion and drawing/thermal pulling | Low cost, time saving (min) | Tip shape limitation | [ | |
Chemical etching | Low cost, reproducibility | Somewhat dangerous | [ | |
High-resolution micromachining (fs laser/focused ion beam/two-photon lithography) | Complex construction designs | Expensive, time-consuming (min–hours) | [ | |
Photo polymeric | Low cost, time-saving (sec–min), good reproducibility | Aging, environmentally unfriendly | [ | |
Microsphere gluing | Low cost, good reproducibility, no contact | Aging | [ |
Table 2. Comparison of Different Fiber Tip Fabrication Methods[40]
Optical fiber end face polishing is a traditional optical fiber micromachining technology, which is usually used in the coupling lens of an optical fiber connector to improve the coupling efficiency of the light beam. The optical fiber end face is formed by grinding and polishing to achieve a large convergence angle. In 1997, Taguchi
Thermal pulling is also a common manufacturing technique for fiber optical tweezers. Generally, the fiber core at the tapered tip becomes smaller, and the output beam is compressed with a large divergence angle, so a particle can be trapped at the fiber tip. We have used an SMF[
Hydrofluoric acid is corrosive to silicon dioxide, so chemical etching can also be used to shape the fiber tip to form fiber optical tweezers. In 2011, Mishra
The preparation methods described are usually limited in their ability to shape microstructures at the optical fiber tip. High-resolution processing methods, such as focused ion beam etching (FIBE) and two-photon polymerization (TPP), can achieve high-resolution processing and manufacturing. In 2006, Cabrini
There are some other methods for fiber optical tweezer fabrication, such as optical polymerization[
2.3. Single-Fiber Taper Optical Tweezers for Yeast Cell Trapping
In the process of the exploration and research of optical fiber tweezers, three-dimensional (3D) optical trapping by single-fiber optical tweezers was first performed in 2006, when Liu
Figure 2.Optical fiber tip tweezers. (a) Parabola-like profile fiber tip; (b) a yeast cell trapped by fiber optical tweezers; (c) and (d) the intensity of the optical field emerging from the fiber tip[
Additional work was inspired by fiber tapering. An SMF was first heated and drawn using a 2–3 nm heating zone with a drawing speed gradually changing from 0.03 to 0.32 mm/s, which led to a diameter decrease from 125 to
To confirm and evaluate the trapping force, a simulation of the beam was performed. The output beam from the fiber tip had a small waist size along with a large divergence angle, which corresponded to a high NA. The output optical field from the tapered fiber tip was simulated, and the results showed that the highest intensity was located at a place
2.4. Microparticle Transfer Between Two Optical Fiber Tweezers
It is convenient to use single optical tweezers to trap microscopic particles, however, the transportation of drug molecules or biological cells is also required in practical applications such as research on the arrangement of microparticles, the interaction between microparticles, and the assembly and testing of nanometer devices. In the field of micromachining, contactless movement, configuration, and assembly in 3D can be realized by optical fiber tweezers, which can achieve accuracy from the micron to the nanoscale level.
Figure
Figure 3.Trapped yeast cell transferred between two optical fiber tweezers. (a) A yeast cell first trapped by a horizontal fiber tip; (b) the yeast cell transferring from the horizontal fiber tip to the vertical fiber tip; (c) the yeast cell transferred to a fiber tip in the vertical direction.
In addition to transferring the particle, the shape of the particle can be adjusted by the two tweezers. This function can be applied in stretching and measuring cellular morphology, which is an indispensable technology in biology.
2.5. Application Example: Microparticle Brownian Motion Force Sensing and Evaluation
The particles to be captured or manipulated are always in a liquid environment. As we all know, the random movement of small particles suspended in a fluid always exists. The interaction of small particles or macromolecules within fluids is a critical issue in microfluidic systems or living cells. Understanding fluid dynamics will help facilitate understanding of the transportation of cells and the effect of small particles on chemical and biological processes. The interaction between particles is weak and difficult to measure. Optical tweezers with the ability to confine the motion of particles can measure the dynamics of a single particle. This feature makes optical tweezers an important tool to investigate the individual behavior and interaction of particles in colloid science. An application of fiber tip tweezers is the measurement for Brownian motion force. Yuan
Figure 4.(a) Brownian motion force evaluating a particle trapped by the fiber tip. (b) The force equilibrium diagram[
At the force equilibrium point, the Brownian motion force can be obtained by measuring the trapping force at the critical point of the particle escaping from the fiber tip by thermal fluctuation. In their experiment, the trapping force was linearly related to the optical power. The motion of the trapped particle in thermal equilibrium is described by the Langevin equation as
In Eq. (
The force includes optical gradient force induced by the optical tweezers, optical scattering force, and thermophoretic force
3. MICRO-OPTICAL HANDS
Micro-optical hand technology is based on optical tweezers. As the optical fiber is flexible and compact, it can act as a probe to investigate the static and dynamic mechanics of the microparticle. Optical tweezers can manipulate the particle as flexibly as human hands.
3.1. Meaning of Micro-Optical Hands
Generally, single optical fiber tweezers can manipulate particles only by trapping and dragging, as opposed to through handover, rotation, and oscillation. For the purpose of manipulating particles as smoothly as the human hand, Yuan’s group did much research and developed particle manipulation technology based on single-fiber tweezers and named these kinds of tweezers ‘micro-optical hands’[
3.2. Multicore Fibers for Making a Micro-Optical Hand
Multicore fibers, especially with annular core distribution, have more advantages for the production of single optical fiber tweezers than traditional SMFs. The multicores can transfer multibeams, which are more convenient, to form focused light beams. The trapping force is formed by the cross combination of the light-field gradient from each core. Then, by adjusting the light power in each core, we can realize complex manipulation of particles including arrangement, rotation, launching, etc. Multiple beams can obtain higher optical power. Generally, twin-core fibers, three-core fibers, four-core fibers, and multicore fibers with circular distribution are suitable for fabricating the single-fiber multicore optical tweezer probe. The advantage of the multicore fiber is that all the cores can bear the same external environment. It not only solves the problem of capturing particles, but also further realizes the adjustment and control of the particle attitude captured by the fiber optical tweezer that can mimic the functionality of a hand.
Like the fabrication of SMF optical tweezers, the fiber end of the multicore fiber also needs to be made into a parabola-like circular truncated cone or spherical-like profile by end polishing technology. The shape of the fiber probe has an influence on the focus of the multiple beams. The grinding angle of the fiber tip can be optimized through theoretical analysis.
3.3. Twin-Core Fiber Optical Hand
The simplest structure of the multicore fiber is the twin-core fiber, and the two cores act as two light channels that can separately transmit light. In 2008, Yuan
Figure 5.Schematic diagram of the optical fiber optical hand concept. (a) Picture of a human hand holding an egg; (b) schematic diagram of a yeast cell controlled by a micro-optical hand built by a multicore fiber.
Figure 6.Twin-core fiber optical tweezers. (a) Cross section of twin-core fiber; (b) abrupt tapered fiber tip; (c) trapped yeast cell by the twin-core fiber tweezers[
The operating distance of the tapered fiber tip is short, which makes the particles very close to the fiber tip. The shape of the tip is a truncated cone. The ground tip can provide a reflecting surface, which is designed for total reflection of the two beams from the two cores to focus on one point. A sufficiently strong gradient force potential well, which is good for trapping particles, was formed at the cross point of the two beams. Figure
Figure 7.Ground tip of the twin-core fiber. (a) A trapped yeast cell at the focus point; (b) a schematic diagram of the transmission beams[
3.4. Three-Core Fiber Optical Hand
As two beams from the twin-core fiber can trap particles, the fiber with multicores distributed circularly is liable to produce focused beams at the output tip. The three beams from the three cores can refract and reflect out of the fiber tip depending on the shape of the fiber end. The output of the three beams from the three cores is combined. The intensity of the light field varies with the focusing position. The maximum intensity is located at the focus point and the intensity decreases, diverging from the focus. Figure
Figure 8.Beam combination field at/out of the focus point. (a) Schematic of a cone-shaped three-core fiber tip; (b) intensity of the output beams; (c) electric-field distribution of the output beams.
3.5. Four-Core Fiber Optical Hand
The four-core fiber has four light waveguide channels that can be used as an optical hand with stronger energy to trap particles. The cross section of the four-core fiber is shown in Fig.
Figure 9.Pyramid end of the four-core fiber for optical hands fabrication[
As introduced above, two beams from the twin-core fibers can generate a trap potential well. The four-core fiber with square distributed cores can form two optical tweezers. The two potential wells can work together to manipulate the particle, achieving the functions of trapping, oscillation, and rotation. Based on the use of four-core fiber, a microparticle transverse harmonic oscillation scheme has been proposed[
3.6. Other Types of Micro-Optical Hands
Based on the concept of a micro-optical hand, another form of optical fiber tweezer can also be called the micro-optical hand. This kind of micro-optical hand adopts different modes of the single-core fiber. Position adjustment of the microparticle occurs through mode division multiplexing technology. The difference in the power of the two modes (
Figure 10.Mode division multiplexing technology based optical tweezers. (a) Experimental setup of the optical tweezers, where the two kinds of SMFs are spliced with a defined offset, and the mode is selected by a fiber micro-bending modulator; (b) position adjustment of the particle by the optical tweezers[
On the basis of the above mentioned single-fiber optical tweezers, Zhang
Figure 11.Single-fiber tweezers for particle adjustment. (a) The truncated cone-shape fiber tip fabricated by the two-step method; (b) microparticle adjusted by the LP11 mode[
A similar case was reported in 2014. Chen
4. OPTICAL GUN
By using a single fiber, a particle can be both 3D trapped and guided, which can be compared to a bullet being loaded and shot. We define this all-fiber particle manipulation device as an optical gun. The fiber used in the optical gun should both possess an annular distribution core and a central core. The light output from the annular distribution core can form a focused ring beam (FRB) that can trap particles. The light shooting from the central core hits the particle to make it escape from the trap point.
4.1. Annular Core Fiber for Trapping
The loaded system in the optical gun uses an annular core fiber. It has the same diameter as traditional fiber that can generate a 3D trapping potential well in front of the fiber tip. The fiber end tip is fabricated into a cone frustum, as shown in Fig.
Figure 12.Annular-core fiber and its cone-frustum tip shape. (a) The cone-frustum end of the annular-core fiber; (b) the cross section of the annular-core fiber; (c) the focus beam from the annular core.
4.2. Add a Push Force: Coaxial Core Fiber
To constitute an optical gun, an additional force should push the particle away after trapping. Thus, a coaxial core fiber (CCF) is made by our lab. The cross section of the fiber end is shown in Fig.
Figure 13.Concept of a fiber optical gun: a coaxial core optical fiber could be used to build a microparticle trapping and shooting system[
4.3. Concept of the Optical Gun
A gun has two functions: loading the bullet and shooting the bullet. The system of integrating both optical trapping and particle shooting can be defined as an optical gun, which mimics the commonly used gun. The all-fiber optical gun is made of a CCF, which consists of a circular core and a central core. The type of optical fiber is not restricted to only one kind. Theoretically, fiber with cores circularly distributed and a central core can constitute the optical gun for both particle trapping and shooting. The fiber tip is usually processed into a cone-frustum shape that is conducive to focusing the output beam. The particle is trapped at the potential well. When a Gaussian beam exits from the central core of the fiber tip, the trapped particle is thrust away along the beam propagation direction, as shown in Fig.
4.4. Coaxial Core Fiber-Based Optical Gun
In 2017, Yuan’s group successfully realized particle trapping and shooting by using a CCF[
Figure 14.Structure of the fiber optical gun. (a) Experimental setup of the fiber optical gun; (b) laser side polishing coupling from the SMF with the annular core of the CCF; (c) the Gaussian beam from the central core and the ring beam from the annular core[
In their experiment, the shooting velocity and shooting length mainly depended on the optical power of the output beam from the central core of the CCF. This device can advantageously perform optical manipulation and particle control, especially in life and medical sciences.
5. OPTICAL FIBER-BASED MULTI PARTICLES TRAPPING AND ARRANGEMENT
In the field of biomedical science and diagnostic systems, the trapping of cell clusters or multiparticles is indispensable. Many researchers have developed optical tweezers toward the application of trapping multiparticles. Yuan’s group demonstrated and analyzed multiparticle trapping using a tapered optical fiber tip tweezer. They theoretically analyzed the multitrapping characteristics using the FDTD method. The schematic diagram of the fiber tip and the photo of the fabricated fiber tip are shown in Figs.
Figure 15.Multiparticle trapping fiber tweezers. (a) The schematic diagram of the fiber-based tweezer; (b) electron microscope image of the etched fiber tip; (c) multiple yeast cells trapped by the optical fiber tweezers.
The effective trapping distance of the tapered-tip single fiber is limited. A stable noninvasive optical trap is necessary in some applications, such as different microparticle comparison tests. Yuan’s group proposed an all-fiber Bessel optical tweezer for multiparticle 3D trapping. By splicing an SMF and a step index MMF, the Bessel beam can be excited at the specially designed output fiber end. The fiber end also employed the “two-step” method: fiber end grinding and discharge current fusion molding. The fiber end of the MMF was fabricated into a special semiellipsoid shape. The experimental setup is shown in Fig.
Figure 16.All-fiber Bessel optical tweezer. (a) The schematic diagram of the experimental setup; (b) the fabricated fiber tip; (c) three cells trapped by the focused Bessel beam[
Optical fiber tweezers can harmlessly manipulate cells
Figure 17.Noncontact optical trapping and arrangement of chloroplasts
6. CONCLUSIONS
A series of function extended fiber optical tweezers have been developed by various specialty optical fibers. Compared with traditional optical tweezers, optical fiber tweezers can be directly inserted into the sample, which enlarges the range of manipulation. They can also be controlled in an initiative way that expands the flexibility and movement dimensions. The capture and observation systems are divided, which results in more degrees of freedom. In addition, the trapping and detecting lights share a single fiber, which is convenient for integration with different systems. However, fiber-based optical tweezers also have drawbacks. The optical fiber is fragile, has low mechanical intensity, and needs coupling/separating technology. In the research of biology, chemistry, and colloids science, optical fiber tweezers are mainly used on the particles with a higher refractive index than the surrounding medium. But, the aqueous systems mostly contain not only high-refractive-index microparticles, but also low-refractive-index microparticles. For trapping the low-refractive-index particles, like air bubbles, a light beam with a hollow intensity distribution, such as doughnut beam, high-order Bessel beams, and other types of hollow beams, is needed[
We named the optical fiber tweezers the “micro-optical hands” and “optical gun” manipulating systems. The fiber-based “micro-optical hands” and “optical gun” manipulating systems are easy to control and conventional to operate as powerful tools for living cell investigation, biology research, life science exploration, and microassembly. Various types of fiber-based optical tweezers are emerging in the fields of biochemistry, cell sorting, and biomedicine and are powerful tools for exploring the microscopic world.
References
[1] A. Ashkin. Phys. Rev. Lett., 24, 156(1970).
[2] A. Ashkin, J. M. Dziedzic. Appl. Phys. Lett., 19, 283(1971).
[3] A. Ashkin, J. M. Dziedzic, J. E. Bjorkholm, S. Chu. Opt. Lett., 11, 288(1986).
[4] Z. Hu, J. Wang, J. Liang. Opt. Express, 12, 4123(2004).
[5] H. Zhang, K. K. Liu. J. R. Soc. Interface, 5, 671(2008).
[6] X. Li, C. Liu, S. Chen, Y. Wang, S. H. Cheng, D. Sun. IEEE Trans. Robot., 33, 1200(2017).
[7] Y. N. Mishra, N. Ingle, S. K. Mohanty. J. Biomed. Opt., 16, 105003(2011).
[8] J. Peřina, J. Ježek, Z. Pilát, M. Šerý, J. Kaňka, O. Samek, S. Bernatová, P. Zemánek, L. Nozka, M. Hrabovský, D. Senderáková, W. Urbańczyk, O. Haderka. Proc. SPIE, 8697, 86970W(2012).
[9] Z. Sun, S. Xu, G. Dai, Y. Li, L. Lou, Q. Liu, R. Zhu. J. Chem. Phys., 119, 2399(2003).
[10] T. Li, S. Kheifets, D. Medellin, M. G. Raizen. Science, 328, 1673(2010).
[12] K. Pearce, F. Wang, P. J. Reece. Opt. Express, 19, 25559(2011).
[13] R. Reimann, M. Doderer, E. Hebestreit, R. Diehl, M. Frimmer, D. Windey, F. Tebbenjohanns, L. Novotny. Phys. Rev. Lett., 121, 033602(2018).
[14] Y. Zhang, P. Liang, Z. Liu, J. Lei, J. Yang, L. Yuan. J. Lightwave Technol., 32, 1394(2014).
[15] A. Ashkin, J. M. Dziedzic, T. Yamane. Nature, 330, 769(1987).
[16] M. C. Zhong, X. B. Wei, J. H. Zhou, Z. Q. Wang, Y. M. Li. Nat. Commun., 4, 1768(2013).
[17] D. G. Grier. Nature, 424, 810(2003).
[18] H. Felgner, O. Muller, M. Schliwa. Appl. Opt., 34, 977(1995).
[19] K. C. Neuman, S. M. Block. Rev. Sci. Instrum., 75, 2787(2004).
[20] J. Liu, Z. Li. Micromachines (Basel), 9, 232(2018).
[21] D. A. White. J. Computat. Phys., 159, 13(2000).
[22] D. A. White. Comput. Phys. Commun., 128, 558(2000).
[23] V. Wong, M. A. Ratner. J. Opt. Soc. Am. B, 23, 1801(2006).
[24] S. H. Simpson, S. Hanna. Opt. Express, 19, 16526(2011).
[25] R. C. Gauthier. Opt. Express, 13, 3707(2005).
[26] W. Sun, S. Pan, Y. Jiang. J. Modern Opt., 53, 2691(2006).
[27] D. C. Benito, S. H. Simpson, S. Hanna. Opt. Express, 16, 2942(2008).
[30] Y. Zhang, Z. Liu, J. Yang, L. Yuan. Proc. SPIE, 8351, 83512X(2012).
[31] J. E. Molloy, M. J. Padgett. Contempor. Phys., 43, 241(2010).
[32] A. Jonás˘, P. Zemánek. Electrophoresis, 29, 4813(2008).
[33] M. Padgett, R. Bowman. Nat. Photon., 5, 343(2011).
[34] C. Pacoret, S. Régnier. Rev. Sci. Instrum., 84, 081301(2013).
[35] H. Su, H. Hu, L. Zhang, X. Ge, Y. Shen. Proc. SPIE, 10154, 101540C(2016).
[36] Y. Tsuboi. Nat. Nanotechnol., 11, 5(2015).
[37] S. R. Lee, J. Kim, S. Lee, Y. Jung, J. K. Kim, K. Oh. Opt. Express, 18, 25299(2010).
[41] Y. Zhang, Z. Liu, J. Yang, L. Yuan. Opt. Commun., 285, 4068(2012).
[42] L. Yuan, Z. Liu, J. Yang, C. Guan. Opt. Express, 16, 4559(2008).
[43] Y. Gong, A.-Y. Ye, Y. Wu, Y.-J. Rao, Y. Yao, S. Xiao. Opt. Express, 21, 16181(2013).
[47] K. Taguchi, H. Ueno, T. Hiramatsu, M. Ikeda. Electron. Lett., 33, 413(1997).
[48] Z. Liu, C. Guo, J. Yang, L. Yuan. Opt. Express, 14, 12510(2006).
[49] Y. Zhang, Z. Liu, J. Yang, L. Yuan. J. Lightwave Technol., 30, 1487(2012).
[52] L. Yuan, Z. Liu, J. Yang. Appl. Phys. Lett., 91, 259(2007).
[53] L. Yuan, Z. Liu, J. Yang, C. Guan. Opt. Express, 16, 4559(2008).
[54] Z. Liu, L. Wang, P. Liang, Y. Zhang, J. Yang, L. Yuan. Opt. Lett., 38, 2617(2013).
[56] S. Chen, H. Huang, H. Zou, Q. Li, J. Fu, F. Lin, X. Wu. J. Opt., 16, 125302(2014).
[58] H. Deng, Y. Zhang, T. Yuan, X. Zhang, Y. Zhang, Z. Liu, L. Yuan. ACS Photon., 4, 642(2017).
[59] P.-B. Liang, J.-J. Lei, Z.-H. Liu, Y. Zhang, L.-B. Yuan. Chin. Phys. B, 23, 088702(2014).
[61] H. Xin, R. Xu, B. Li. Laser Photon. Rev., 7, 801(2013).
[62] Y. Li, H. Xin, X. Liu, B. Li. Sci. Rep., 5, 10925(2015).
[63] F. Peng, B. Yao, S. Yan, W. Zhao, M. Lei. J. Opt. Soc. Am. B, 26, 2242(2009).
[64] G. Rui, Y. Wang, X. Wang, B. Gu, Y. Cui. J. Phys. Commun., 2, 065015(2018).
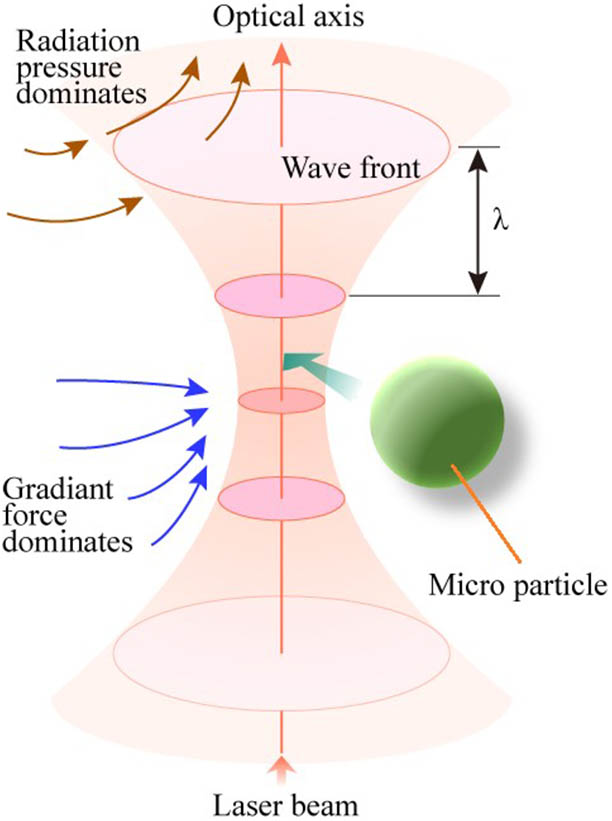
Set citation alerts for the article
Please enter your email address