Lithium niobate on insulator (LNOI), as an emerging and promising optical integration platform, faces shortages of on-chip active devices including lasers and amplifiers. Here, we report the fabrication of on-chip erbium-doped LNOI waveguide amplifiers based on electron beam lithography and inductively coupled plasma reactive ion etching. A net internal gain of ~30 dB/cm in the communication band was achieved in the fabricated waveguide amplifiers under the pump of a 974 nm continuous laser. This work develops new active devices on LNOI and may promote the development of LNOI integrated photonics.

- Chinese Optics Letters
- Vol. 19, Issue 6, 060008 (2021)
Abstract
1. Introduction
As an excellent optical crystal material, lithium niobate (
Another important part of integrated optical systems is the active LNOI devices, such as lasers and amplifiers. It is known that LN is an indirect band gap material emitting light inefficiently. The luminescence of LN can be achieved with the help of doping rare earth ions. Recently, some researchers first prepared waveguide and microring structures on LNOIs using electron beam lithography (EBL) and dry etching processes, and then doped them with erbium and ytterbium ions by ion implantation followed by thermal annealing at ∼500°C to repair the lattice damage. Based on these rare-earth-ions-doped LNOI devices, photoluminescence experiments were carried out[
The on-chip LNOI amplifier, as a fundamental optical component of active LNOI devices, however, is rarely reported. Here, we present the fabrication of LNOI waveguide amplifiers using EBL and inductively coupled plasma reactive ion etching (ICP-RIE) processes on homemade erbium-doped LNOI wafers. Under the 980 nm band pump, a net internal gain of ∼15 dB at 1531.5 nm was realized on a ∼5-mm-long waveguide structure. The dynamic behavior of gain from linear to saturation was observed in the cases of both fixed signals with increasing pump power and fixed pump power with increasing signal power.
Sign up for Chinese Optics Letters TOC. Get the latest issue of Chinese Optics Letters delivered right to you!Sign up now
2. Fabrication of Erbium-Doped LNOI Waveguide
Fabrication of erbium-doped waveguide amplifiers starts from an erbium-doped X-cut LNOI wafer with a doping concentration of 0.1% (mole fraction). The wafer is composed of a 600-nm-thick erbium-doped LN film that was sliced from a homemade LN crystal wafer, a 2-µm-thick silicon dioxide (
Figure 1.(a) Schematic fabrication processes of erbium-doped LNOI waveguides. (b) Optical micrograph of fabricated erbium-doped LNOI waveguides. (c) Simulation results of mode distributions regarding fundamental transverse electric modes at wavelengths of 974 nm (top) and 1532 nm (bottom).
3. Characterizations of Erbium-Doped LNOI Waveguide Amplifiers
Respecting the higher absorption coefficient of erbium ions in the 980 nm band compared with that in the 1480 nm band[
Figure 2.Experimental setup for gain characterization in erbium-doped LNOI waveguide amplifiers. VOA, variable optical attenuator; OC, optical coupler; PM, power meter; PC, polarization controller; WDM, wavelength division multiplexer; OSA, optical spectrum analyzer. The photograph of the erbium-doped LNOI chip clearly shows the generated green fluorescence propagating along the straight waveguide.
To calibrate the net internal gain of erbium-doped LNOI waveguide amplifiers, we first characterized the optical propagation losses for both the pump and signal of chip waveguides using whispering-gallery-resonator-loss measurements based on an erbium-doped microring resonator coupling with the waveguide structure on the same chip. As shown in Fig. 3, through fitting the resonance spectrum by a Lorentz function, the load
Figure 3.Optical transmission spectra of Er-doped LNOI microring resonators on the same chip in (a) the 1550 nm band and (b) the 980 nm band. The Lorentz fit (red line) showing
The net internal gain of the erbium-doped LNOI waveguide amplifier was defined as
Figure 4.Gain characterization in erbium-doped LNOI waveguide amplifiers. (a) The dependence of net internal gain on pump power at a fixed signal power of ∼5 nW. (b) Measured signal spectra at ∼1531.47 nm with increasing pump powers of 0, 0.10 mW, 7.35 mW, 16.19 mW, 32.31 mW, and 64.02 mW. (c) The net internal gain as a function of increasing signal power at fixed pump power of ∼23 mW. (d) The amplified spontaneous emission (ASE) spectrum of the erbium-doped LNOI waveguide at pump power of 0.57 mW.
Moreover, we characterized the gain dependence of an erbium-doped LNOI waveguide amplifier on the signal power. As shown in Fig. 4(c), at low signal power, the net internal gain decreases linearly with the increasing signal power for a fixed pump power of ∼23 mW, corresponding to the small signal gain state (−65 dBm to −50 dBm). As the signal power continues to increase, gain saturation is observed. The maximum gain is ∼15 dB (∼30 dB/cm) with the signal power of ∼65 dBm, and there is still ∼0.13 dB amplification at ∼34 dBm signal power. As shown in the amplified spontaneous emission (ASE) spectrum of the erbium-doped LNOI waveguide with pump power of 0.57 mW [Fig. 4(d)], although the optimal working wavelength is ∼1531.5 nm, the amplifier works in a wide range of the communication band.
4. Conclusions
In summary, we fabricated on-chip erbium-doped LNOI waveguide amplifiers using EBL and ICP-RIE processes. Under the 980 nm band pump, the communication band amplifiers were demonstrated with maximum net internal gain of ∼15 dB achieved in a 5-mm-long chip. Compared with the previous
During the preparation of this article, we noticed that two researches on erbium-doped waveguide amplifiers were posted on arXiv[
References
[1] Y. Kong, F. Bo, W. Wang, D. Zheng, H. Liu, G. Zhang, R. Rupp, J. Xu. Recent progress in lithium niobate: optical damage, defect simulation, and on-chip devices. Adv. Mater., 32, 1806452(2020).
[2] J. Lin, F. Bo, Y. Cheng, J. Xu. Advances in on-chip photonic devices based on lithium niobate on insulator. Photon. Res., 8, 1910(2020).
[3] M. Zhang, C. Wang, R. Cheng, A. Shams-Ansari, M. Loncar. Monolithic ultra-high-Q lithium niobate microring resonator. Optica, 4, 1536(2017).
[4] R. Wu, M. Wang, J. Xu, J. Qi, W. Chu, Z. Fang, J. Zhang, J. Zhou, L. Qiao, Z. Chai, J. Lin, Y. Cheng. Long low-loss-lithium niobate on insulator waveguides with sub-nanometer surface roughness. Nanomaterials, 8, 910(2018).
[5] R. Gao, H. Zhang, F. Bo, W. Fang, Z. Hao, N. Yao, J. Lin, J. Guan, L. Deng, M. Wang, L. Qiao, Y. Cheng. Ultrahigh quality-factor microresonators fabricated in pristine lithium niobate thin film for efficient nonlinear optics applications(2021).
[6] Z. Hao, J. Wang, S. Ma, W. Mao, F. Bo, F. Gao, G. Zhang, J. Xu. Sum-frequency generation in on-chip lithium niobate microdisk resonators. Photon. Res., 5, 623(2017).
[7] Z. Hao, L. Zhang, A. Gao, W. Mao, X. Lyu, X. Gao, F. Bo, F. Gao, G. Zhang, J. Xu. Periodically poled lithium niobate whispering gallery mode microcavities on a chip. Sci. Chin. Phys. Mech. Astron., 61, 114211(2018).
[8] C. Wang, C. Langrock, A. Marandi, M. Jankowski, M. Zhang, B. Desiatov, M. M. Fejer, M. Lončar. Ultrahigh-efficiency wavelength conversion in nanophotonic periodically poled lithium niobate waveguides. Optica, 5, 1438(2018).
[9] J.-Y. Chen, Z.-H. Ma, Y. M. Sua, Z. Li, C. Tang, Y.-P. Huang. Ultra-efficient frequency conversion in quasi-phase-matched lithium niobate microrings. Optica, 6, 1244(2019).
[10] J. Lu, J. B. Surya, X. Liu, A. W. Bruch, Z. Gong, Y. Xu, H. X. Tang. Periodically poled thin-film lithium niobate microring resonators with a second-harmonic generation efficiency of 250,000%/W. Optica, 6, 1455(2019).
[11] J. Lin, N. Yao, Z. Hao, J. Zhang, W. Mao, M. Wang, W. Chu, R. Wu, Z. Fang, L. Qiao, W. Fang, F. Bo, Y. Cheng. Broadband quasi-phase-matched harmonic generation in an on-chip monocrystalline lithium niobate microdisk resonator. Phys. Rev. Lett., 122, 173903(2019).
[12] Z. Hao, L. Zhang, W. Mao, A. Gao, X. Gao, F. Gao, F. Bo, G. Zhang, J. Xu. Second-harmonic generation using d33 in periodically poled lithium niobate microdisk resonators. Photon. Res., 8, 311(2020).
[13] L. Zhang, Z. Hao, Q. Luo, A. Gao, R. Zhang, C. Yang, F. Gao, F. Bo, G. Zhang, J. Xu. Dual-periodically poled lithium niobate microcavities supporting multiple coupled parametric processes. Opt. Lett., 45, 3353(2020).
[14] C. Wang, M. Zhang, X. Chen, M. Bertrand, A. Shams-Ansari, S. Chandrasekhar, P. Winzer, M. Lončar. Integrated lithium niobate electro-optic modulators operating at CMOS-compatible voltages. Nature, 562, 101(2018).
[15] M. He, M. Xu, Y. Ren, J. Jian, Z. Ruan, Y. Xu, S. Gao, S. Sun, X. Wen, L. Zhou, L. Liu, C. Guo, H. Chen, S. Yu, L. Liu, X. Cai. High-performance hybrid silicon and lithium niobate Mach–Zehnder modulators for 100 Gbit s−1 and beyond. Nat. Photon., 13, 359(2019).
[16] Y. He, Q.-F. Yang, J. Ling, R. Luo, H. Liang, M. Li, B. Shen, H. Wang, K. Vahala, Q. Lin. Self-starting bi-chromatic LiNbO3 soliton microcomb. Optica, 6, 1138(2019).
[17] Z. Gong, X. Liu, Y. Xu, M. Xu, J. B. Surya, J. Lu, A. Bruch, C. Zou, H. X. Tang. Soliton microcomb generation at 2 µm in z-cut lithium niobate microring resonators. Opt. Lett., 44, 3182(2019).
[18] M. Zhang, B. Buscaino, C. Wang, A. Shams-Ansari, C. Reimer, R. Zhu, J. M. Kahn, M. Loncar. Broadband electro-optic frequency comb generation in a lithium niobate microring resonator. Nature, 568, 373(2019).
[19] D. Pohl, M. Reig Escalé, M. Madi, F. Kaufmann, P. Brotzer, A. Sergeyev, B. Guldimann, P. Giaccari, E. Alberti, U. Meier, R. Grange. An integrated broadband spectrometer on thin-film lithium niobate. Nat. Photon., 14, 24(2020).
[20] S. Wang, L. Yang, R. Cheng, Y. Xu, M. Shen, R. L. Cone, C. W. Thiel, H. X. Tang. Incorporation of erbium ions into thin-film lithium niobate integrated photonics. Appl. Phys. Lett., 116, 151103(2020).
[21] D. Pak, H. An, A. Nandi, X. Jiang, Y. Xuan, M. Hosseini. Ytterbium-implanted photonic resonators based on thin film lithium niobate. J. Appl. Phys., 128, 084302(2020).
[22] S. Dutta, E. A. Goldschmidt, S. Barik, U. Saha, E. Waks. Integrated photonic platform for rare-earth ions in thin film lithium niobate. Nano Lett., 20, 741(2020).
[23] Z. Wang, Z. Fang, Z. Liu, W. Chu, Y. Zhou, J. Zhang, R. Wu, M. Wang, T. Lu, Y. Cheng. On-chip tunable microdisk laser fabricated on Er3+-doped lithium niobate on insulator. Opt. Lett., 46, 380(2021).
[24] Y. Liu, X. Yan, J. Wu, B. Zhu, Y. Chen, X. Chen. On-chip erbium-doped lithium niobate microcavity laser. Sci. Chin. Phys. Mech. Astron., 64, 234262(2021).
[25] Q. Luo, Z. Hao, C. Yang, R. Zhang, D. Zheng, S. Liu, H. Liu, F. Bo, Y. Kong, G. Zhang, J. Xu. Microdisk lasers on an erbium-doped lithium-niobite chip. Sci. Chin. Phys. Mech. Astron., 64, 234263(2021).
[26] C. Huang, L. McCaughan. 980-nm-pumped Er-doped LiNbO3 waveguide amplifiers: a comparison with 1484-nm pumping. IEEE J. Sel. Top. Quantum Electron, 2, 367(1996).
[27] R. Brinkmann, I. Baumann, M. Dinand, W. Sohler, H. Suche. Erbium-doped single- and double-pass Ti:LiNbO3 waveguide amplifiers. IEEE J. Quantum Electron., 30, 2356(1994).
[28] H. Chi-Hung, L. McCaughan. Er-indiffused Ti:LiNbO3 channel waveguide optical amplifiers pumped at 980 nm. Electron. Lett., 32, 215(1996).
[29] S. Suntsov, C. E. Rüter, D. Kip. Er:Ti:LiNbO3 ridge waveguide optical amplifiers by optical grade dicing and three-side Er and Ti in-diffusion. Appl. Phys. B, 123, 118(2017).
[30] D. Brüske, S. Suntsov, C. E. Rüter, D. Kip. Efficient ridge waveguide amplifiers and lasers in Er-doped lithium niobate by optical grade dicing and three-side Er and Ti in-diffusion. Opt. Express, 25, 29374(2017).
[31] J. Zhou, Y. Liang, Z. Liu, W. Chu, H. Zhang, D. Yin, Z. Fang, R. Wu, J. Zhang, W. Chen, Z. Wang, Y. Zhou, M. Wang, Y. Cheng. On-chip integrated waveguide amplifiers on erbium-doped thin film lithium niobate on insulator(2021).
[32] Z. Chen, Q. Xu, K. Zhang, W.-H. Wong, D.-L. Zhang, E. Y.-B. Pun, C. Wang. Efficient erbium-doped thin-film lithium niobate waveguide amplifiers(2021).
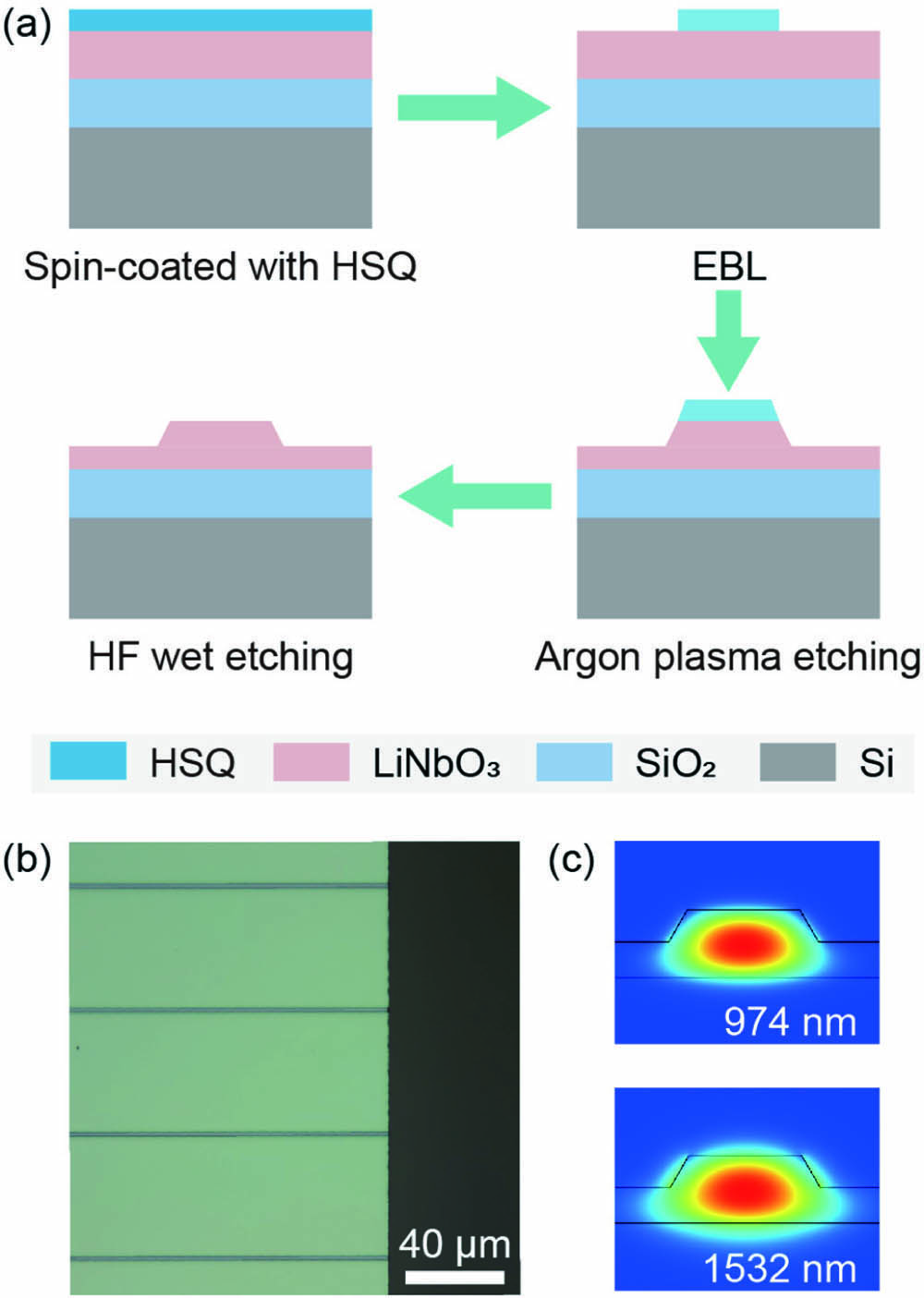
Set citation alerts for the article
Please enter your email address