Abstract
Gold nanorods (GNRs) with two different aspect ratios were successfully utilized as saturable absorbers (SAs) in a passively Q-switched neodymium-doped lutetium lithium fluoride (Nd:LLF) laser emitting at 1.34 μm. Based on the GNRs with an aspect ratio of five, a maximum output power of 1.432 W was achieved, and the narrowest pulse width was 328 ns with a repetition rate of 200 kHz. But, in the case of the GNRs with the aspect ratio of eight, a maximum output power of 1.247 W was achieved, and the narrowest pulse width was 271 ns with a repetition rate of 218 kHz. Our experimental results reveal that the aspect ratios of GNRs have different saturable absorption effects at a specific wavelength. In other words, for passively Q-switched lasers at a given wavelength, we are able to select the most suitable GNRs as an SA by changing their aspect ratio.The appearance of the pulsed laser has attracted worldwide attention since it has many practical and potential applications in the fields of optical machining, remote sensing, medical treatment, telecommunications, and source pumping[1–3]. Passive -switching technology as one of the most effective methods to generate a pulsed laser has received increasing interest because of its simple structure. For this kind of laser, the performance mainly depends on the properties of the saturable absorber (SA), which may modulate the intracavity loss periodically. Traditional SAs, such as -doped yttrium aluminum garnet (), GaAs, , and V:YAG, have been widely used in passively -switched lasers[4–7]. In recent years, with the advent of graphenes, two-dimensional (2D) materials like black phosphorus, , , and have drawn attention of some researchers[8–11]. One of the main advantages of 2D materials is their broadband absorption spectrum due to the unique band gap structure.
Besides the materials mentioned above, anisotropic metal nanoparticles can also be used as SAs because of their ultrafast nonlinear optical response[12]. Among all the anisotropic metal nanoparticles that have been employed, gold nanorods (GNRs) are the most stable ones, and they present some fascinating aspects, such as the assembly of multiple types involving materials science, the behavior of the individual particles, and size-related electronic, magnetic, and optical properties[13–15]. Compared with traditional SAs and 2D materials, the main advantage of using GNRs is that the position of the absorption peak can be controlled by adjusting the aspect ratio (length/diameter) of GNRs[16–18]. This shape-dependent property allows GNRs to be applied in -switched lasers with different wavelengths. One striking feature in the UV-visible (vis)-near-infrared (NIR) absorption spectrum of a solution of GNRs is that there are two absorption peaks [one peak for the transverse (short axis) plasmon and the other for the longitudinal (long axis) plasmon] caused by surface plasmon resonance (SPR), which is an optical phenomenon that occurs when a nanoparticle of an appropriate size interacts with incident photons in such a way as to confine the resonant photon within the dimensions of the nanoparticle[16,19,20]. Besides, the position of the absorption peak of GNRs’ longitudinal SPR is aspect ratio dependence rather than length dependence[21]. In addition, the absorption peak caused by the longitudinal SPR can be tuned from the middle of the vis region of the electromagnetic spectrum () to the NIR region () by altering the aspect ratio of the GNRs[16,18]. Furthermore, Elim et al. reported their observation of reverse-saturable and saturable absorption at SPR in the longitudinal direction in GNRs[20]. All these features indicate that GNRs have the potential to become an excellent SA.
Recently, with GNRs as an SA, passive -switching solid-state lasers ranging from 0.6 to 2 μm were reported[22–27]. GNRs have been proven to be used as successful SAs. However, they did not mention the influence of different aspect ratios on the output of the -switching laser.
Sign up for Chinese Optics Letters TOC. Get the latest issue of Chinese Optics Letters delivered right to you!Sign up now
Herein, we investigate a diode-end-pumped passively -switched neodymium-doped lutetium lithium fluoride (Nd:LLF) laser at 1.34 μm, which has not been demonstrated before, by utilizing the GNRs with two different aspect ratios as SAs. For the laser utilizing the GNRs with an aspect ratio of five (GNR-5) as an SA, the narrowest pulse width was 328 ns with a repetition rate of 200 kHz. The maximum value of the average output power and single pulse energy were 1.432 W and 7.2 μJ, respectively. To the best of our knowledge, this is the first time that the maximum value of average output power can exceed 1.4 W for a diode-end-pumped solid-state laser with GNRs as SAs. While for a laser utilizing the GNRs with an aspect ratio of eight (GNR-8), the narrowest pulse width was 271 ns with a repetition rate of 218 kHz.
The GNRs samples in our experiments were synthesized through a seed-mediated growth method[17]. The first step was to prepare the seed solution. In brief, 5 mL of 0.5 mmol/L solution was added into 5 mL of 0.2 mol/L hexadecyl trimethyl ammonium bromide (CTAB) solution. Then, 0.6 mL of 0.01 mol/L ice-cold solution was mixed with the prepared solution under vigorous stirring conditions. It was found that at the beginning the color of the solution was yellow, and it changed into tawny in a few seconds. After the resulting mixed solution was stirred for 2 min and then kept at 30°C for 30 min, the seed solution was successfully prepared. The next step was to prepare two kinds of growth solutions for the GNRs with aspect ratios of five and eight, respectively. Specifically, the growth solution for the GNR-8 was prepared via the following procedures. First, 0.95 g CTAB, 0.11 g sodium 5-bromo-2-hydroxybenzoate, and 25 mL of deionized water were mixed in a flask at 60°C. Secondly, when the mixed solution was naturally cooled to 30°C, 3.0 mL of 4 mmol/L was added to the flask, and the resulting mixed solution was kept at 30°C for 15 min. Then, 25 mL of 1 mmol/L and 0.42 mL of 12 mol/L HCl were added to the flask, and the mixed solution was slowly stirred for 15 min. Finally, 0.125 mL of 0.064 mol/L ascorbic acid was added to the above mixed solution under strong stirring, until the solution became colorless and transparent. The growth solution for the GNR-5 was prepared by following similar procedures, but HCl no longer needed to be added. Besides, the additional amount of 4 mmol/L was reduced to 2.4 mL, and the additional amount of 0.064 mol/L ascorbic acid was increased to 0.2 mL. When the growth solution was prepared, 0.08 mL well-dispersed seed solution was transferred into it, and then the resulting final solution was mixed thoroughly and kept at 30°C for 12 h. In this way, the GNR solution was obtained.
A transmission electron microscope (TEM, JEOL JEM 2100) was used to observe the size and shape of the prepared GNRs. Figures 1(a) and 1(b) show the size, shape, and morphology of two kinds of GNRs with different aspect ratios. The length and width of GNRs in Fig. 1(a) are about 40 and 8 nm, where the aspect ratio is five, while the length and width of GNRs in Fig. 1(b) are about 50 and 6 nm, where the aspect ratio is eight. It is important to note that several spheroidal particles exist among the GNRs [see Fig. 1(b)]. Seeing that the absorption peak of spheroidal gold nanoparticles is located at about 500 nm, far from the longitudinal absorption peak of GNR-8, the possible influence of spheroidal particles on the saturable absorption property can be ignored.
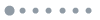
Figure 1.TEM images of the (a) GNR-5 and (b) GNR-8.
The as-prepared GNR solution was dropped onto flat silicon and then dried at room temperature (about 20°C) for at least 12 h to form the GNR films that apply to our laser system. At first, the absorption spectra of the GNR solutions, which were recorded by a UV-vis-NIR spectrophotometer (U-4100 Hitachi), were investigated. The recorded spectra were shown in Fig. 2(a). It is observed from Fig. 2(a) that the GNR-5 showed two isolated absorption peaks. The small SPR peak near 520 nm is due to the transverse mode perpendicular to the GNRs; the second SPR peak near 800 nm is due to the length area of the GNRs, which gives rise to the longitudinal mode of SPR with a higher peak.
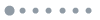
Figure 2.(a) Absorption spectra and (b) nonlinear transmissions of the GNRs with two aspect ratios.
Similar to GNR-5, GNR-8 also shows a peak near 520 nm, which is caused by the transverse SPR. The larger peak near 1200 nm originates from the longitudinal SPR. Besides, an additional absorption peak is observed at about 430 nm from the absorption spectrum. It is possible that the residual ions were reduced to Ag particles in the GNR solution.
For purpose of testing the saturable absorption property of GNRs, we utilized a , 90 kHz -switched laser at 1340 nm as a light source. As shown in Fig. 2(b), the nonlinear transmission of GNRs at 1340 nm increases gradually along with input pulse fluence. The modulation depth and the saturated transmission of GNR-5 were 16% and 96%, respectively, while the modulation depth and the saturated transmission of GNR-8 were 11% and 91%. These results suggest that both GNR-5 and GNR-8 have saturable absorption property at 1340 nm.
Our results confirm that, according to the absorption spectra and nonlinear transmissions, the absorption behavior of the GNR samples can be influenced by their aspect ratio.
The setup of our experiments was demonstrated in Fig. 3. The pump source was a commercial fiber-coupled laser diode working at 794 nm. The fiber core was 200 μm in diameter, and its numerical aperture (NA) was 0.22. The light from the pump source was reimaged into the laser crystal with radius of 100 μm by a coupling system. The resonant cavity was composed of a concave mirror (M1) with a curvature radius of 150 mm and a plane mirror (M2), where the distance between them was 30 mm. In addition, M1 was anti-reflection (AR) coated at 794 nm and 1.05 μm and high-reflection (HR) coated at 1.34 μm. M2 was employed as an output coupling mirror. The high-quality [100]-cut Nd:LLF crystal with -doped concentration of 1.0 at. % was used as the gain medium. In order to prevent the crystal from overheating, the crystal was enclosed with indium foil in Cu blocks, and we used a water-cooling system to keep the Cu blocks at 18°C. Besides, two end faces of the crystal were polished and AR-coated at 794 nm and 1.34 μm.
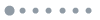
Figure 3.Scheme of diode-end-pumped passively -switched laser.
Two output couplers (OCs) with transmissions of 3.8% and 8% at 1340 nm were employed, respectively. The power of the output laser was measured by a PM100D power meter with an S314C power head (Thorlabs Inc., USA). Figure 4(a) shows the continuous-wave (CW) output power without any SA being inserted into the resonator cavity. The maximum value of output power, which was obtained by utilizing the OC of , was 1.846 W, and the slope efficiency was 18.7%. With an OC of being employed, a maximum power of 1.709 W was achieved with a slope efficiency of 17.5%. The measured data of the beam quality factor () of the CW laser beam at the maximum output power are shown in Fig. 4(b), which was obtained by a 90%/10% scanning knife-edge method. As can be seen from Fig. 4(b), the optimum fitting results of the factor were 1.36 in the tangential direction and 1.32 in the sagittal direction, respectively. The small difference between the tangential and sagittal directions was caused by the aberration when the beam propagated in the device. The factor did not have a distinct difference between the 3.8% and 8% OCs.
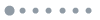
Figure 4.(a) Output power and (b) factor of the CW laser with the 3.8% and 8% OCs.
Firstly, the performance of GNR-5 was investigated. After GNR-5 was inserted into the cavity, the thresholds of the pump laser were 5.32 and 6.2 W for the 3.8% and 8% OCs, respectively. Stable -switching operations were realized when incident pump power ranged from 5.32 to 9.6 W and 6.2 to 9.96 W for the 3.8% and 8% OCs. At incident pump powers of 9.6 and 9.96 W, the output power reached 1.432 W for the 3.8% OC and 1.173 W for the 8% OC. If the incident pump power became higher than 9.6 and 9.96 W, the -switching operation became unstable. In the case of utilizing GNR-5 as an SA, the average output power as a function of the incident pump power was shown in Fig. 5(a). The maximum value of average output power got lower as compared with CW output power, which might be caused by two reasons: (1) the inserting loss of the GNR SA; (2) the reflection loss on the surface due to the uncoated substrate. As can be seen in Fig. 5(b), the central wavelength was 1339.96 nm, measured by a commercial spectrum analyzer (MS3504i, made in Belarus).
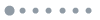
Figure 5.(a) Output power of the -switched laser with the 3.8% and 8% OCs; (b) spectrum of the passively -switched laser when utilizing GNR-5 as an SA.
The pulse temporal behavior, including pulse width and pulse repetition rate, was recorded by a digital oscilloscope (1 GHz bandwidth and 20 GSamples/s sampling rate, DPO 7401 C Tektronix Inc., USA) and a fast pin photodiode detector with a rise time of 0.4 ns. We fetched the data of the pulse width and the repetition rate from the screen of the digital oscilloscope at the same time. Under the situation of utilizing the 3.8% OC, the relationships between pulse width, pulse repetition rate, and incident pump power are exhibited in Fig. 6(a). The minimum pulse width was 328 ns at a 200 kHz pulse repetition rate, with a single pulse energy of 7.2 μJ. To the best of our knowledge, the 7.2 μJ single pulse energy is much higher than the previous results obtained in the diode-end-pumped GNR -switched laser[22,23] because its average output power is one order of magnitude higher than those achieved in Refs. [22,23]. When the 8% OC was utilized, the minimum pulse width was 460 ns at a 205 kHz pulse repetition rate, corresponding to a single pulse energy of 5.7 μJ. The pulse width declined from 1247 to 460 ns with the pulse repetition rate ascending from 72 to 205 kHz, as shown in Fig. 6(c).
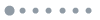
Figure 6.(a) Pulse width and repetition rate with the 3.8% OC; (b) waveform of the -switched laser utilizing GNR-5 with the 3.8% OC; (c) pulse width and repetition rate with the 8% OC; (d) waveform of the -switched laser utilizing GNR-5 with the 8% OC.
The waveforms of 328 ns with the 3.8% OC and 460 ns with the 8% OC were depicted in Figs. 6(b) and 6(d). The fluctuation of the height of the waveform was less than 5% in 1 h, so we can draw a conclusion that the pulsed laser output is very stable. If the GNR film was removed, -switched pulses would not be observed any more. Thus, we can confirm that -switched pulses were generated by the saturable absorption of GNRs.
The investigation method of GNR-8 is similar to that of GNR-5. We also chose two mirrors with the transmissions of 3.8% and 8% at 1340 nm as OCs. The relationship between average output power and incident pump power is depicted in Fig. 7(a). We can see that the maximum average output powers were about 1.209 W with the 3.8% OC and 1.247 W with the 8% OC. Meanwhile, as depicted in Fig. 7(b), the central wavelength was 1340.06 nm.
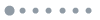
Figure 7.(a) Output power of the -switched laser versus the incident pump power with the 3.8% and 8% OCs; (b) spectrum of the passively -switched laser utilizing GNR-8 as an SA.
The dependence of the pulse width and pulse repetition rate on the pump power was shown in Fig. 8(a) (for 3.8% OC) and Fig. 8(c) (for 8% OC), respectively. As the incident pump power rose, the pulse width decreased, while the pulse repetition rate increased. When utilizing the 3.8% OC, the pulse width ranged from 1824 to 271 ns as the incident pump power rose. Meanwhile, the pulse repetition rate changed from 71 to 218 kHz. Under the maximum average output power and pulse repetition rate, the single pulse energy was 5.6 μJ, and the waveform was shown in Fig. 8(b). When utilizing the 8% OC, the narrowest pulse width was 438 ns with 227 kHz, as depicted in Fig. 8(d), corresponding to single pulse energy of 5.4 μJ. The pulsed laser output using GNR-8 as an SA was as stable as the pulsed laser output using GNR-5 as an SA.
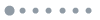
Figure 8.(a) Pulse width and repetition rate with the 3.8% OC; (b) waveform of the passively -switched laser utilizing GNR-8 as an SA with the 3.8% OC; (c) pulse width and repetition rate with the 8% OC; (d) waveform of the passively -switched laser utilizing GNR-8 as an SA with the 8% OC.
By comparing the output laser performance of two different SAs, we can find that the pulse width obtained with GNR-8 as an SA is relatively narrower. We believe the possible reason is that the center wavelength of the absorption spectrum of GNR-8 is closer to 1340 nm, as shown in Fig. 2(a). So, GNR-8 is a more suitable SA for the -switched Nd:LLF laser at 1.34 μm compared with GNR-5.
By employing two different aspect ratio GNRs, diode-end-pumped passively -switched Nd:LLF lasers at 1.34 μm were successfully realized. The narrowest pulse width of 271 ns was achieved with pulse repetition rate of 218 kHz by utilizing GNR-8 and the 3.8% OC. Our work indicates that GNRs with different aspect ratios have different saturable absorption effects at a specific wavelength. Therefore, by controlling the aspect ratio, GNRs can be used as a kind of suitable SA for a -switched laser covering a wide wavelength range from vis to infrared.
References
[1] A. Okhotnikov, M. Grudinin, M. Pessa. New J. Phys., 6, 177(2004).
[2] U. Keller, D. A. B. Miller, G. D. Boyd, T. H. Chiu, J. F. Ferguson, M. T. Asom. Opt. Lett., 17, 505(1992).
[3] Y. Zhang, Z. Wei, B. Zhou, C. Xu, Y. Zou, D. Li, Z. Zhang, H. Zhang, J. Wang, H. Yu, K. Wu, B. Yao, J. Wang. Opt. Lett., 34, 3316(2009).
[4] J. Dong. Opt. Commun., 226, 337(2003).
[5] M. Malyarevich, I. A. Denisov, K. V. Yumashev, V. P. Mikhailov, R. S. Conroy, B. D. Sinclair. Appl. Phys. B, 67, 555(1998).
[6] W. W. Ge, H. J. Zhang, J. Y. Wang, X. F. Cheng, M. H. Jiang, C. L. Du, S. C. Yuan. Opt. Express, 13, 3883(2005).
[7] J. Yang, Q. Fu, J. Liu, Y. Wang. Laser Phys. Lett., 4, 20(2007).
[8] B. Zhang, F. Lou, R. Zhao, J. He, J. Li, X. Su, J. Ning, K. Yang. Opt. Lett., 40, 3691(2015).
[9] H. Liu, A. Luo, F. Wang, R. Tang, M. Liu, Z. Luo, W. Xu, C. Zhao, H. Zhang. Opt. Lett., 39, 4594(2014).
[10] C. Janisch, N. Mehta, D. Ma, A. L. Elias, N. Perea-Lopez, M. Terrones, Z. Liu. Opt. Lett., 39, 383(2014).
[11] B. Bai, Y. Bai, D. Li, Y. Sun, J. Li, J. Bai. Chin. Opt. Lett., 16, 031402(2018).
[12] H. Baida, D. Mongin, D. Christofilos, G. Bachelier, A. Crut, P. Maioli, N. Del Fatti, F. Vallee. Phys. Rev. Lett., 107, 057402(2011).
[13] M. C. Daniel, D. Astruc. Chem. Rev., 104, 293(2004).
[14] L. Vigderman, B. P. Khanal, E. R. Zubarev. Adv. Mater., 24, 4811(2012).
[15] S. Link, C. Burda, M. B. Mohamed, B. Nikoobakht, M. A. El-Sayed. Phys. Rev. B, 61, 6086(2000).
[16] S. E. Lohse, C. J. Murphy. Chem. Mater., 25, 1250(2013).
[17] M. Grzelczak, J. Perez-Juste, P. Mulvaney, L. M. Liz-Marzan. Chem. Soc. Rev., 37, 1783(2008).
[18] S. Link, M. A. El-Sayed, M. B. Mohamed. J. Phys. Chem. B, 109, 10531(1999).
[19] H. Chen, L. Shao, Q. Li, J. Wang. Chem. Soc. Rev., 42, 2679(2013).
[20] H. I. Elim, J. Yang, J. Y. Lee, J. Mi, W. Ji. Appl. Phys. Lett., 88, 083107(2006).
[21] X. Ye, L. Jin, H. Caglayan, J. Chen, G. Xing, C. Zheng, D. Vicky, Y. Kang, N. Engheta, C. R. Kagan, C. B. Murray. ACS Nano, 6, 2804(2012).
[22] T. Song, C. Feng, X. Chen, Q. Wang, G. Qin, W. Qin, X. Gao, Y. Dun, P. Li. Laser Phys. Lett., 14, 055808(2017).
[23] C. Feng, M. Liu, Y. Li, X. Gao, Z. Kang, G. Qin, J. Jia, X. Tao, T. Song, Y. Dun, F. Bai, P. Li, Q. Wang, J. Fang. Appl. Phys. B, 123, 81(2017).
[24] H. Zhang, B. Li, J. Liu. Appl. Opt., 55, 26(2016).
[25] D. Wu, J. Peng, Z. Cai. Opt. Express, 23, 24071(2015).
[26] H. Huang, M. Li, P. Liu, L. Jin, H. Wang, D. Shen. Opt. Lett., 41, 2700(2016).
[27] G. Zhang, T. Liu, Y. Shen, C. Zhao, B. Huang, Z. Kang, G. Qin, Q. Liu, X. Fu. Chin. Opt. Lett., 16, 020011(2018).