Abstract
We demonstrate a harmonically pumped femtosecond optical parametric oscillator (OPO) laser using a frequency-doubled mode-locked Yb:KGW laser at a repetition rate of 75.5 MHz as the pump laser. Based on a bismuth borate nonlinear crystal, repetition rates up to 1.13 GHz are realized, which is 15 times that of the pump laser. The signal wavelength is tunable from 700 nm to 887 nm. The maximum power of the signal is 207 mW at the central wavelength of 750 nm and the shortest pulse duration is 117 fs at 780 nm. The beam quality (M2 factor) in the horizontal and vertical directions of the output beam are 1.077 and 1.141, respectively.Broadly tunable ultrafast laser sources in the spectral range of the visible and near infrared at GHz repetition rates play an important role in the fields of nonlinear optics and time-resolved spectroscopy[1,2]. With the rapid development of femtosecond laser technology and the birth of some new nonlinear crystals, tunable wavelengths are widely obtained based on optical parametric amplifier (OPA)[3–6], difference frequency generation (DFG)[7], sum frequency generation[8], and optical parametric oscillators (OPOs)[9]. Compared to OPA and DFG, femtosecond OPO could realize broadly tunable signal and idler synchronously by utilizing a mode-locked femtosecond oscillator at a high repetition rate. Due to the fact that the cavity length of the OPO can be shortened to fractions of that of the pump laser, which can be expressed as (where N is an integer), the repetition rate of the signal pulses can be extended to N times that of the pump laser. The higher repetition rate of the signal means a shorter OPO cavity length. The signal pulses need to travel an integer number of round trips in the OPO cavity, which results in an increase of the pumping threshold of OPO. In 1997, Reid et al. reported the first harmonically pumped femtosecond OPO using a rubidium titanyl arsenate (RTA) crystal, and the repetition rate of the signal was 344 MHz corresponding to four times that of the pump source[10]. In 2000, Phillips et al. reported a 322 MHz femtosecond OPO using a periodically poled lithium niobate (PPLN) crystal, the repetition rate of which was four times that of the pump source. The output signals had a power of 280 mW and achieved a tunable wavelength range from 1 μm to [11]. Two years later, Jiang et al. reported a GHz-level harmonically pumped OPO, corresponding to 12 times that of the pump source[12]. It is worth pointing out that all of the high-repetition-rate OPOs above are pumped by mode-locked Ti:sapphire femtosecond lasers, so that the signal wavelength is usually tunable from to . In the past few years, with the development of Yb-doped ultrafast lasers using solid state crystal, fiber, and thin disks, it shows a superior performance for pumping a femtosecond OPO due to its high stability and beam quality[13–17]. In 2012, Lang et al. reported a noncollinear OPO (NOPO) using a barium borate (BBO) crystal that is pumped by a 22 W frequency-doubled thin disk laser[18]. The signal wavelength was tuned from 680 nm to 850 nm and the maximum power of the signal was 3 W. In 2014, Gu et al. reported a dual wavelength fs OPO based on a lithium triborate (LBO) crystal pumped by a frequency-doubled Yb fiber laser[19]. In 2018, Fan et al. reported a harmonically pumped 1 GHz fs OPO based on a compact V-type cavity, and the pump source was a Yb fiber oscillator[20]. After one year, they further reported a 910 MHz harmonically pumped OPO, which was pumped by a 101 MHz fiber oscillator[21]. The signal wavelength was tuned in a range from 1350 nm to 1610 nm and the maximum signal power was 1.04 W. Although there has been a lot of progress of high repetition rate OPOs in the NIR and IR wavelength range, high-repetition rate OPOs up to the GHz level harmonically pumped by a frequency-doubled Yb fs laser are less reported. Such a device that can provide a high-repetition-rate femtosecond laser source in the spectral range of the visible and near infrared has not been reported.
In this Letter, we report a 1.13 GHz femtosecond bismuth borate (BIBO) OPO system harmonically pumped by a commercial frequency-doubled mode-locked Yb:KGW laser. A ring cavity OPO with a cavity length of 26.5 cm is arranged using a 1.5 mm long BIBO as a nonlinear crystal; the repetition rate of 1.13 GHz corresponds to 15 times that of the pump laser. The signal wavelength is tuned from 700 nm to 887 m. The maximum output power is 207 mW at the central wavelength of 750 nm, and the shortest pulse duration is 117 fs at 780 nm. The factors in the horizontal and vertical directions are 1.077 and 1.141, respectively.
The experimental setup of the high-repetition-rate OPO is shown in Fig. 1. The central wavelength of the pump laser at 515 nm is generated from a frequency-doubled commercial mode-locked Yb:KGW laser (Light Conversion, FLINT6.0). The Yb:KGW laser delivers an average power of 7 W at 1030 nm with a pulse duration of 100 fs. By frequency doubling using a 2.5 mm long LBO crystal, the average power of the 515 nm laser is 3.3 W, corresponding to the fundamental to SHG efficiency of 47%. The repetition rate of the pump laser is 75.5 MHz, corresponding to a cavity length of 1986 mm. Two pieces of dichroic mirrors (high transmission at 1030 nm, high reflection at 515 nm) are used to separate the 515 nm laser and the fundamental laser. A half-wave plate (HWP1) and a polarizing beam splitter (PBS) are employed to change the pump power by rotating the polarization of the pump laser[22]. HWP2 is used to change the polarization direction of the pump laser from horizontal to vertical. The pump laser is focused into BIBO crystal by a focal lens (L1) with a focal length of 100 mm. The OPO has a ring cavity configuration that is composed of a pair of concave chirped mirrors (CM1 and CM2) with a radius of curvature of 50 mm, one plane mirror (M1), and an output coupler (OC). The concave mirrors are highly reflective for the wavelength range of 700 nm to 1000 nm, and each of them contains a group delay dispersion (GDD) for compensating intracavity positive dispersion. In addition, concave mirrors CM1 and CM2 are high transmission coated () for the pump laser at 488–532 nm. M1 and OC are highly reflective in the range of 650 nm to 1050 nm. The OPO cavity length is 26.5 cm, which corresponds to the repetition rate of 1.13 GHz, 15 times that of the pump laser. The signal will travel 15 times in the OPO cavity to match the pump pulse temporally, so an OC with a transmission of 3% is used for decreasing the cavity loss. A 1.5 mm long BIBO crystal is selected as the nonlinear crystal due to its large effective nonlinear coefficient. Table 1 lists the comparison of of different nonlinear crystals used in the 515 nm laser pumped OPO. The BIBO crystal is cut at , for type I () phase matching in the Y−Z plane, and both end faces are high anti-reflection coated in the spectral range of 510−520 nm and 650−1050 nm.
Sign up for Chinese Optics Letters TOC. Get the latest issue of Chinese Optics Letters delivered right to you!Sign up now
Crystal | BBO | LBO (x-y plane) | BIBO (y-z plane) |
---|
deff (pm/V) | 2.03 | 0.838 | 2.86 |
Table 1. Comparison of the deff for different nonlinear crystals (515 nm→750 nm+1643.6 nm)
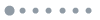
Figure 1.Experimental setup for the 1.13 GHz ring cavity OPO. DM1,2: dichroic mirror, HWP1,2: half-wave plate, PBS: polarizing beam splitter, L1: lens of , CM1, CM2: concave spherical mirrors, M1: plane mirror, OC: 3% output coupler.
The OPO cavity is aligned by the pump laser. The first signal wavelength of 720 nm is extracted from the OC when the OPO cavity length satisfies the harmonically pumped condition. The output power of the signal at 720 nm is measured under different pump powers, as shown in Fig. 2(a). The maximum signal power is 190 mW under the pump power of 3.3 W. The high pump threshold is 3 W due to the high loss of harmonically pumped OPO. The wavelength of the signal is tunable from 700 to 887 nm by rotating the phase-matching angle of the BIBO crystal. The maximum signal power is 207 mW at the central wavelength of 750 nm under the pump power of 3.3 W, corresponding to the pump to signal conversion efficiency of 6.3%. There are two reasons for the decrease of the average power with the increase of the signal wavelength. First, tuning the signal spectrum to a longer wavelength requires a larger incident angle on the BIBO crystal, so the spatial coupling condition of the pump and the signal will get worse. Second, the longer signal wavelength approaches the degenerate point (1030 nm), at which the signal power will be the lowest. Therefore, the average power of the signal decreases when the spectrum is tuned to a longer wavelength. The minimum signal power at 887 nm is just 3 mW, as shown in Fig. 2(b), and the wavelength could be tuned to 1000 nm with more pump power available.
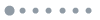
Figure 2.(a) Output power of the signal at 720 nm versus the pump power, and (b) the normalized signal tuning range and the corresponding average power.
Figures 3(a) and 3(b) show the pump pulse trains and signal pulse trains, respectively. Both of them are detected by a fast photodiode and recorded by an oscilloscope with 1 GHz bandwidth (DPO5104B, Tektronix). Obviously, there are 15 signal pulses in the period of the pump pulse. It is clear that the intensity of the first signal pulse is greater than that of the subsequent 14 pulses. The intensity of the signal pulse trains shows a downward trend in pump period because the latter signal pulses suffer one more cavity round-trip loss than the previous ones. The signal pulse trains are also detected by a 26 GHz radio-frequency spectrum analyzer (R&S FSW26). Figure 3(c) exhibits the radio-frequency (RF) spectrum of the 1.13 GHz signal pulses, spanning 2.5 GHz with a resolution bandwidth of 1 MHz. The 1.13 GHz signal reveals a signal-to-noise ratio of 77 dB at the fundamental beat note and the side mode suppression ratio is about 24 dB. The clean RF spectrum makes clear that the OPO cavity is well synchronous with that of the pump source. Except for the 1.13 GHz repetition frequency, the other harmonic frequencies also exist since the intensity of the signal pulse trains has a periodic modulation at the periods of 13.2 ns, i.e., 75.5 MHz. This is consistent with the repetition rate of the pump source.
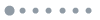
Figure 3.(a) Pulse train of the pump laser, (b) pulse train of the 1.13 GHz signal pulses, which is 15 times that of the pump laser, and (c) the RF spectrum of the 1.13 GHz signal laser at a 2.5 GHz span with a 1 MHz resolution.
For compensation of the OPO intracavity positive group delay dispersion (GDD) introduced by the BIBO crystal and the air, a pair of concave chirped mirrors (CM1 and CM2) were used in the OPO cavity. The pulse duration of the different signal wavelengths is measured by using an intensity autocorrelator, and the shortest pulse duration is 117 fs at the central wavelength of 780 nm assuming a Gaussian profile, as shown in Fig. 4(a), which is approximately the transform-limited pulse duration 112 fs calculated from the measured signal laser spectrum. There are 15 signal pulses in one pump period. The latter signal pulses suffer one more cavity net GDD. Consider the and GDD induced by the BIBO and the air at the central wavelength of 780 nm, respectively. The net dispersion inside the OPO cavity is about at 780 nm. We can calculate that the pulse durations of two adjacent pulses are nearly identical based on the equation of . The difference between the pulse durations of the first and 15th signal pulse in one pump period is 13 fs. Figure 4(b) shows the beam spatial profile () of the 1.13 GHz signal laser measured by an factor meter (Spiricon M2–200s. The factors in the horizontal and vertical directions are 1.077 and 1.141, respectively.
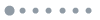
Figure 4.(a) Measured signal pulse temporal profile at 780 nm and corresponding spectrum, and (b) measured spatial profile of a 1.13 GHz signal laser.
The phase noise power spectral density (PSD) of the fundamental 1.13 GHz signal is measured by a commercial spectrum analyzer (R&S FSW26). As shown in Fig. 5, the phase noise of the signal pulses is mainly from cavity length fluctuations. The frequency-resolved phase noise from 1 Hz to 1 kHz reveals a high phase noise, which is attributed to the vibration of the laboratory optical platform and air agitation. It should be noted that our OPO is not isolated by a baffle and the cavity length is unlocked by the circuit system. Lower phase noise is expected in this frequency range if the laboratory conditions are more stable and the OPO cavity length is actively locked. As a contrast, the signal pulses exhibit a low noise performance () in the range of 1 kHz to 10 MHz, which benefits from the high performance of the pump laser. The calculated integrated phase noise (IPN) from 1 Hz to 10 MHz is merely 79.8 mrad, which is also shown in Fig. 5 (black curve).
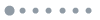
Figure 5.Red curve, phase noise PSD of the signal from 1 Hz to 10 MHz. Blue curve, phase noise PSD of the pump from 1 Hz to 10 MHz. Black curve, IPN of the signal from 1 Hz to 10 MHz.
In conclusion, a high-repetition-rate femtosecond OPO is demonstrated that is harmonically pumped by a frequency-doubled mode-locked Yb:KGW laser. A ring cavity OPO with a cavity length of 26.5 cm is realized using a 1.5 mm long BIBO crystal with a repetition rate of 1.13 GHz, which corresponds to 15 times that of the pump laser. The signal wavelength is tunable from 700 nm to 887 nm. The maximum signal power is 207 mW at 750 nm, and the shortest pulse duration is 117 fs at 780 nm. The factors in the horizontal and vertical directions are 1.077 and 1.141, respectively. The signal average power can be scalable to over 1 W with a higher power pump source and has potential applications in ultrafast nonlinear optics and time-resolved spectroscopy.
References
[1] T. Sekikawa, A. Kosuge, T. Kanai, S. Watanabe. Nature, 432, 605(2004).
[2] A. Bartels, T. Dekorsy, H. Kurz. Opt. Lett., 24, 996(1999).
[3] A. M. Siddiqui, G. Cirmi, D. Brida, F. X. Kartner, G. Cerullo. Opt. Lett., 34, 3592(2009).
[4] M. Baudisch, B. Wolter, M. Pullen, M. Hemmer, J. Biegert. Opt. Lett., 41, 3583(2016).
[5] S. A. Rezvani, Z. F. Hong, X. X. Pang, S. Wu, Q. B. Zhang, P. X. Lu. Opt. Lett., 42, 3367(2017).
[6] X. Liang, X. L. Xie, C. Zhang, J. Kang, Q. W. Yang, P. Zhu, A. L. Guo, H. D. Zhu, S. H. Yang, Z. R. Cui, M. Z. Sun, J. Q. Zhu. Opt. Lett., 43, 5713(2018).
[7] J. Darginavicius, G. Tamosauskas, A. Piskarskas, G. Valiulis, A. Dubietis. Appl. Phys. B, 108, 1(2012).
[8] Y. Guan, F. Wang, Y. Yang, D. Wang, X. Zhang, Q. Yuan, W. Zhou, D. X. Hu, X. W. Deng, H. J. Ren. Chin. Opt. Lett., 17, 081401(2019).
[9] D. C. Edelstein, E. S. Wachman, C. L. Tang. Appl. Phys. Lett., 54, 1728(1989).
[10] D. T. Reid, C. McGowan, W. Sleat, M. Ebrahimzadeh, W. Sibbett. Opt. Lett., 22, 525(1997).
[11] P. J. Phillips, S. Das, M. Ebrahimzadeh. Appl. Phys. Lett., 77, 469(2000).
[12] J. Jiang, T. Hasama. Appl. Phys. B, 74, 313(2002).
[13] W. L. Tian, Z. H. Wang, X. H. Meng, N. H. Zhang, J. F. Zhu, Z. Y. Wei. Opt. Lett., 41, 4851(2016).
[14] W. L. Tian, Z. H. Wang, J. F. Zhu, Z. Y. Wei. Opt. Express, 24, 29815(2016).
[15] N. Coluccelli, D. Viola, V. Kumar, A. Perri, M. Marangoni, G. Cerullo, D. Polli. Opt. Lett., 42, 4545(2017).
[16] P. Liu, Z. W. Zhang. Opt. Lett., 43, 4735(2018).
[17] Y. X. Ma, F. Meng, Y. Wang, A. M. Wang, Z. G. Zhang. Chin. Opt. Lett., 17, 111001(2019).
[18] T. Lang, T. Binhammer, S. Rausch, G. Palmer, M. Emons, M. Schultze, A. Harth, U. Morgner. Opt. Express, 20, 912(2012).
[19] C. L. Gu, M. L. Hu, J. T. Fan, Y. J. Song, B. W. Liu, C. Y. Wang. Opt. Lett., 39, 3896(2014).
[20] J. T. Fan, Y. X. Chu, H. S. Shi, J. Zhao, L. Chai, C. Y. Wang, M. L. Hu. IEEE Photonics Technol. Lett., 30, 2159(2018).
[21] Y. X. Chen, J. T. Fan, W. K. Yang, J. Zhao, Y. X. Chu, M. L. Hu. Opt. Lett., 44, 1638(2019).
[22] X. H. Meng, Z. H. Wang, J. W. Jiang, W. L. Tian, S. B. Fang, Z. Y. Wei. J. Opt. Soc. Am. B, 35, 967(2018).