
- Chinese Optics Letters
- Vol. 21, Issue 4, 042701 (2023)
Abstract
Keywords
1. Introduction
Quantum photonic technology exhibits great potential for applications ranging from quantum communications[1–3] and quantum computation[4–6] to quantum metrology[7,8]. In particular, the recent advances in integrated quantum photonics[9–13] have shown great promise for chip-scale quantum information processing with unprecedented capability and complexity. Single-mode, high-purity, and integrated sources of single photons and/or entangled photon pairs are necessary for all these quantum protocols[14–16].
Sign up for Chinese Optics Letters TOC. Get the latest issue of Chinese Optics Letters delivered right to you!Sign up now
Silicon photonic integration platforms provide third-order nonlinearity
The traditional fabrication method for QPM is electric field poling, but it cannot be used to manipulate
2. Device Structure Fabrication and Characteristics
In our experiment, the LN channel waveguide is fabricated by diffusing a 35-nm-thick titanium layer with a width of 3 µm on the
Figure 1.Simulated mode profiles of the quasi-TM-polarized mode (a) at 812 nm for the downconverted photons and (b) at 406 nm for the pump. The scale bar is 2 µm.
The experimental setup for the periodic inversion of ferroelectric domains is shown in Fig. 2(a). The laser (central wavelength at 800 nm, pulse width 180 fs, repetition rate 76 MHz, and single pulse energy up to 5 nJ) for domain inversion of the waveguide is generated by a femtosecond oscillator (MIRA, Coherent). The diameter of the focus spot on the crystal surface is about 1 µm by using a
Figure 2.(a) Experimental setup for femtosecond laser direct writing ferroelectric domain patterns in the Ti-indiffused LN channel waveguide. HWP, half-wave plate; PBS, polarizing beam splitter; DM, dichroic mirror; IF, interference filter. (b) Optical microscopic image of the 2D optically poled domain pattern with the period of 2.74 µm in the x direction and 1.15 µm in the y direction. The inverted domains are visible as small circles. (c) 3D profiles of the inverted domains obtained by Cerenkov second-harmonic microscopy.
An optimal quantum source should be able to produce a large number of photon pairs, which is determined by the nonlinear conversion efficiency of the periodically poled waveguide. For this reason, it is crucial to characterize both the propagation losses of light and nonlinear conversion efficiency to optimize the parameters for the SPDC. We will use classical characterization of the device to predict quantum behavior because the generation and detection of classical light are easier than for quantum light. First we study the reverse process of parametric downconversion (PDC), that is, second-harmonic generation (SHG). We use the microscopic objective of
Figure 3.Measured output intensity distribution of (a) the fundamental and (b) the second-harmonic waves in the far field; spectrum for (c) the fundamental and (d) the second-harmonic waves; (e) normalized output power of the second harmonic versus the input polarization of the fundamental wave at temperature 30°C; (f) normalized conversion efficiency of second harmonic versus the quasi-phase-matching temperature.
3. Experimental Results
The photons produced by the SPDC are measured to characterize the performance of our periodically poled LN waveguide. The experimental setup for the quantum measurements is shown in Fig. 4(a). The phase-matching condition for the SPDC was already obtained from the SHG measurements before. A 406 nm pump is generated from the SHG of the 812 nm femtosecond laser (Chameleon, Coherent) using the BBO crystal. We use two short-pass filters (Thorlabs FESH0450) and a bandpass filter (10 nm bandwidth centered 405 nm) to block the 812 nm photons. The device temperature is stabilized at 30°C. We use a polarization controller to adjust the polarization of the pump to the TM mode, which is required for Type-0 SPDC. Then, the microscopic objective (C280TMD-A) is used to focus the 406 nm laser beam into the 10 mm LN waveguide poled by the femtosecond laser, and the emitted photons are collected using the microscope objective (RMS 4X-PF). The generated signal and idler photons at 812 nm with the same vertical polarization are separated by a 50:50 fiber coupler and detected by single-photon detectors with detection efficiencies of 80%. In order to measure only the downconverted photon pairs, the emerging photons from the waveguide pass through two long-pass filters (FELH0700) and a bandpass filter (10 nm bandwidth centered at 810 nm) for blocking the 406 nm photons.
Figure 4.(a) Schematic of the experimental setup used for photon-pair measurements. SMF, single-mode fiber; FC, fiber coupler; SPD, single-photon detector; M, mirror. (b) The coincidence of photon pairs varies with the average pump power.
The coincidence rate
4. Conclusion
In conclusion, we have demonstrated all-optical fabrication of QPM structures in Ti-indiffused LN waveguide using a femtosecond laser, which is a one-step process without masking procedure. The proposed scheme is efficient enough to produce correlated photon pairs based on the SPDC. The generated coincidence rate can reach ∼8000 counts per second for an average pump power of 3.2 mW. Our results indicate that the femtosecond laser poling provides a powerful and flexible platform for fabricating periodic ferroelectric domains, which will benefit quantum photonic applications. It is anticipated that the manipulations of complex quantum states will be available by employing the femtosecond laser poling to control the 3D ferroelectric domains.
References
[1] S. Pirandola, U. L. Andersen, L. Banchi, M. Berta, D. Bunandar, R. Colbeck, D. Englund, T. Gehring, C. Lupo, C. Ottaviani, J. L. Pereira, M. Razavi, J. Shamsul Shaari, M. Tomamichel, V. C. Usenko, G. Vallone, P. Villoresi, P. Wallden. Advances in quantum cryptography. Adv. Opt. Photonics, 12, 1012(2020).
[2] J. Yin, Y.-H. Li, S.-K. Liao, M. Yang, Y. Cao, L. Zhang, J.-G. Ren, W.-Q. Cai, W.-Y. Liu, S.-L. Li, R. Shu, Y.-M. Huang, L. Deng, L. Li, Q. Zhang, N.-L. Liu, Y.-A. Chen, C.-Y. Lu, X.-B. Wang, F. Xu, J.-Y. Wang, C.-Z. Peng, A. K. Ekert, J.-W. Pan. Entanglement-based secure quantum cryptography over 1,120 kilometres. Nature, 582, 501(2020).
[3] J.-Y. Hu, B. Yu, M.-Y. Jing, L.-T. Xiao, S.-T. Jia, G.-Q. Qin, G.-L. Long. Experimental quantum secure direct communication with single photons. Light Sci. Appl., 5, e16144(2016).
[4] J. M. Arrazola, V. Bergholm, K. Bradler, T. R. Bromley, M. J. Collins, I. Dhand, A. Fumagalli, T. Gerrits, A. Goussev, L. G. Helt, J. Hundal, T. Isacsson, R. B. Israel, J. Izaac, S. Jahangiri, R. Janik, N. Killoran, S. P. Kumar, J. Lavoie, A. E. Lita, D. H. Mahler, M. Menotti, B. Morrison, S. W. Nam, L. Neuhaus, H. Y. Qi, N. Quesada, A. Repingon, K. K. Sabapathy, M. Schuld, D. Su, J. Swinarton, A. Száva, K. Tan, P. Tan, V. D. Vaidya, Z. Vernon, Z. Zabaneh, Y. Zhang. Quantum circuits with many photons on a programmable nanophotonic chip. Nature, 591, 54(2021).
[5] J. B. Spring, B. J. Metcalf, P. C. Humphreys, W. S. Kolthammer, X.-M. Jin, M. Barbieri, A. Datta, N. Thomas-Peter, N. K. Langford, D. Kundys, J. C. Gates, B. J. Smith, P. G. R. Smith, I. A. Walmsley. Boson sampling on a photonic chip. Science, 339, 798(2012).
[6] H.-S. Zhong, H. Wang, Y.-H. Deng, H.-S. Zhong, H. Wang, Y.-H. Deng, M.-C. Chen, L.-C. Peng, Y.-H. Luo, J. Qin, D. Wu, X. Ding, Y. Hu, P. Hu, X.-Y. Yang, W.-J. Zhang, H. Li, Y. Li, X. Jiang, L. Gan, G. Yang, L. You, Z. Wang, L. Li, N.-L. Liu, C.-Y. Lu, J.-W. Pan. Quantum computational advantage using photons. Science, 370, 1460(2020).
[7] M. A. Taylor, W. P. Bowen. Quantum metrology and its application in biology. Phys. Rep., 615, 1(2011).
[8] V. Giovannetti, S. Lloyd, L. Maccone. Advances in quantum metrology. Nat. Photonics, 5, 222(2011).
[9] J.-W. Wang, F. Sciarrino, A. Laing, M. G. Thompson. Integrated photonic quantum technologies. Nat. Photonics, 14, 273(2020).
[10] L.-L. Lu, X.-D. Zheng, Y.-Q. Lu, S.-N. Zhu, X.-S. Ma. Advances in chip-scale quantum photonic technologies. Adv. Mater. Technol., 4, 2100068(2021).
[11] J. Kim, S. Aghaeimeibodi, J. Carolan, D. Englund, E. Waks. Hybrid integration methods for on-chip quantum photonics. Appl. Phys. Lett., 7, 291(2020).
[12] C. P. Dietrich, A. Fiore, M. G. Thompson, M. Kamp, S. Hofling. GaAs integrated quantum photonics: towards compact and multi-functional quantum photonic integrated circuits. Laser Photonics Rev., 10, 870(2016).
[13] L.-T. Feng, M. Zhang, J.-W. Wang, X.-Q. Zhou, X.-G. Qiang, G.-C. Guo, X. Ren. Silicon photonic devices for scalable quantum information applications. Photonics Res., 10, A135(2022).
[14] P. Senellart, G. Solomon, A. White. High-performance semiconductor quantum-dot single-photon sources. Nat. Nanotechnol., 12, 1026(2017).
[15] Y.-C. Wang, K. D. Jons, Z.-P. Sun. Integrated photon-pair sources with nonlinear optics. Appl. Phys. Rev., 8, 11314(2021).
[16] L. Caspani, C.-L. Xiong, B. J. Eggleton, D. Bajoni, M. Liscidini, M. Galli, R. Morandotti, D. J. Moss. Integrated sources of photon quantum states based on nonlinear optics. Light Sci. Appl., 6, e17100(2017).
[17] A. Orieux, M. A. M. Versteegh, K. D. Jons, S. Ducci. Semiconductor devices for entangled photon pair generation: a review. Rep. Prog. Phys., 80, 76001(2017).
[18] X.-Y. Lu, S. Rogers, T. Gerrits, W. C. Jiang, S. Nam, Q. Lin. Heralding single photons from a high-q silicon microdisk. Optica, 3, 1331(2016).
[19] Z.-H. Yin, K. Sugiura, H. Takashima, R. Okamoto, F. Qiu, S. Yokoyama, S. Takeuchi. Frequency correlated photon generation at telecom band using silicon nitride ring cavities. Opt. Express, 29, 4821(2021).
[20] S. Castelletto, A. Peruzzo, C. Bonato, B. C. Johnson, M. Radulaski, H.-Y. Ou, F. Kaiser, J. Wrachtrup. Silicon carbide photonics bridging quantum technology. ACS Photonics, 9, 1434(2022).
[21] Z. Ma, J. Chen, Z. Li, C. Tang, Y. M. Sua, H. Fan, Y.-P. Huang. Ultrabright quantum photon sources on chip. Phys. Rev. Lett., 125, 263602(2020).
[22] Z.-D. Xie, T. Zhong, S. Shrestha, X.-A. Xu, J.-L. Liang, Y.-X. Gong, J. C. Bienfang, A. Restelli, J. H. Shapiro, F. N. C. Wong, C. W. Wong. Harnessing high-dimensional hyperentanglement through a biphoton frequency comb. Nat. Photonics, 9, 536(2015).
[23] S. Bogdanov, M. Y. Shalaginov, A. Boltasseva, V. M. Shalaev. Material platforms for integrated quantum photonics. Opt. Mater. Express, 7, 111(2017).
[24] B.-Y. Xu, L.-K. Chen, J.-T. Lin, L.-T. Feng, R. Niu, Z.-Y. Zhou, R.-H. Gao, C.-H. Dong, G.-C. Guo, Q.-H. Gong, Y. Cheng, Y.-F. Xiao, X.-F. Ren. Spectrally multiplexed and bright entangled photon pairs in a lithium niobate microresonator. Sci. China Phys. Mech. Astron., 65, 294262(2022).
[25] T. Wang, P. Chen, C. Xu, Y. Zhang, D.-Z. Wei, X.-P. Hu, G. Zhao, M. Xiao, S. Zhu. Periodically poled LiNbO3 crystals from 1D and 2D to 3D. Sci. China Technol., 63, 1110(2020).
[26] J. Zhao, C.-X. Ma, M. Rusing, S. Mookherjea. High quality entangled photon pair generation in periodically poled thin-film lithium niobate waveguides. Phys. Rev. Lett., 124, 163603(2020).
[27] H. Jin, F. M. Liu, P. Xu, J. L. Xia, M. L. Zhong, Y. Yuan, J. W. Zhou, Y. X. Gong, W. Wang, S. N. Zhu. On-chip generation and manipulation of entangled photons based on reconfigurable lithium-niobate waveguide circuits. Phys. Rev. Lett., 113, 103601(2014).
[28] M. B. Nasr, S. Carrasco, B. E. A. Saleh, A. V. Sergienko, M. C. Teich, J. P. Torres, L. Torner, D. S. Hum, M. M. Fejer. Ultrabroadband biphotons generated via chirped quasi-phase-matched optical parametric down-conversion. Phys. Rev. Lett., 100, 183601(2008).
[29] A. Tanaka, R. Okamoto, H. Lim, S. Subashchandran, M. Okano, L.-B. Zhang, L. Kang, J. Chen, P. Wu, T. Hirohata, S. Kurimura, S. Takeuchi. Noncollinear parametric fluorescence by chirped quasi-phase matching for monocycle temporal entanglement. Opt. Express, 20, 25228(2012).
[30] D. Wei, C. Wang, H. Wang, X. Hu, D. Wei, X. Fang, Y. Zhang, D. Wu, Y. Hu, J. Li, S. Zhu, M. Xiao. Experimental demonstration of a three dimensional lithium niobate nonlinear photonic crystal. Nat. Photonics, 12, 596(2018).
[31] T.-X. Xu, K. Switkowski, X. Chen, S. Liu, K. Koynov, H.-H. Yu, H. Zhang, J. Wang, Y. Sheng, W. Krolikowski. Three-dimensional nonlinear photonic crystal in ferroelectric barium calcium titanate. Nat. Photonics, 12, 591(2018).
[32] Y. Zhang, Y. Sheng, S.-N. Zhu, M. Xiao, W. Krolikowski. Nonlinear photonic crystals: from 2D to 3D. Optica, 8, 372(2021).
[33] B. Zhang, L. Wang, F. Chen. Recent advances in femtosecond laser processing of LiNbO3 crystals for photonic applications. Laser Photonics Rev., 14, 1900407(2020).
[34] X.-Y. Xu, T.-X. Wang, P.-C. Chen, C. Zhou, J.-N. Ma, D.-Z. Wei, H. Wang, B. Niu, X. Fang, D. Wu, S. Zhu, M. Gu, M. Xiao, Y. Zhang. Femtosecond laser writing of lithium niobate ferroelectric nanodomains. Nature, 609, 496(2022).
[35] X.-P. Hu, Y. Zhang, S.-N. Zhu. Nonlinear beam shaping in domain engineered ferroelectric crystals. Adv. Mater., 32, 1903775(2019).
[36] D.-Z. Wei, C.-W. Wang, X.-Y. Xu, H.-J. Wang, Y.-L. Hu, P.-C. Chen, J. Li, Y. Zhu, C. Xin, X. Hu, Y. Zhang, D. Wu, J. Chu, S. Zhu, M. Xiao. Efficient nonlinear beam shaping in three dimensional lithium niobate nonlinear photonic crystals. Nat. Commun., 10, 4193(2019).
[37] S. Liu, K. Switkowski, C.-L. Xu, J. Tian, B.-X. Wang, P.-X. Lu, W. Krolikowski, Y. Sheng. Nonlinear wavefront shaping with optically induced three-dimensional nonlinear photonic crystals. Nat. Commun., 10, 3208(2019).
[38] B.-X. Wang, S. Liu, T.-X. Xu, R.-W. Zhao, P.-X. Lu, W. Krolikowski, Y. Sheng. Nonlinear talbot self-healing in periodically poled LiNbO3 crystal. Opt. Express, 19, 60011(2021).
[39] S. Liu, L. M. Mazur, W. Krolikowski, Y. Sheng. Nonlinear volume holography in 3D nonlinear photonic crystals. Laser Photonics Rev., 14, 2000224(2020).
[40] P.-C. Chen, C.-W. Wang, D.-Z. Wei, Y.-L. Hu, X.-Y. Xu, J.-W. Li, D. Wu, J. Ma, S. Ji, L. Zhang, L. Xu, T. Wang, C. Xu, J. Chu, S. Zhu, M. Xiao, Y. Zhang. Quasi-phase-matching-division multiplexing holography in a three-dimensional nonlinear photonic crystal. Light Sci. Appl., 10, 146(2021).
[41] Q. Yu, C. Xu, S.-X. Chen, P.-C. Chen, S.-W. Nie, S.-J. Ke, D. Wei, M. Xiao, Y. Zhang. Manipulating orbital angular momentum entanglement in three-dimensional spiral nonlinear photonic crystals. Photonics, 9, 504(2022).
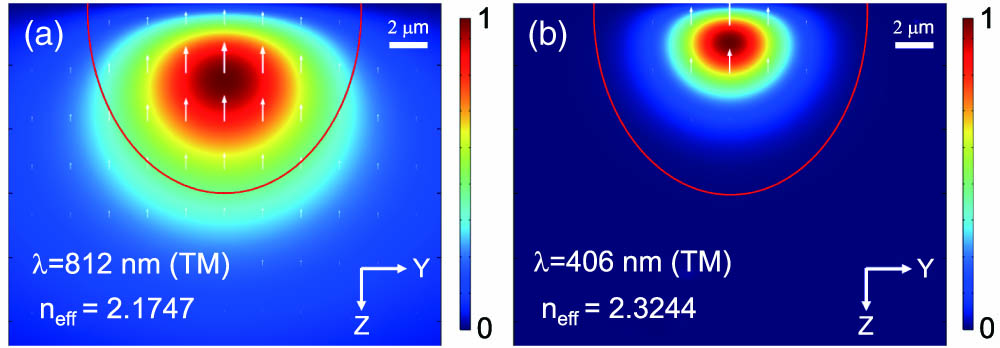
Set citation alerts for the article
Please enter your email address