V. M. Romanova*, A. R. Mingaleev, A. E. Ter-Oganesyan, T. A. Shelkovenko, G. V. Ivanenkov, and S. A. Pikuz
Abstract
The results of experiments with rapidly exploding thin conductors in the current-pause regime are presented. Copper wires 25 μm in diameter and 12 mm in length serve as loads for a GVP pulsed generator based on a low-inductance capacitor. The generator produces current pulses of up to 10 kA with dI/dt up to 50 A/ns. A 100–800-ns current-pause regime is obtained for charging voltages of 10–15 kV. The discharge channel structure is studied by shadow photography using 0.53-μm, 10-ns second-harmonic pulses from a Nd3+:YAG laser. In the experiments, three types of secondary breakdown are observed, with different symmetry types, different current-pause durations, and different dependences on the energy deposited into the wire during its resistive heating. All of these breakdown types develop inside a tubular core that is produced in the current-pause stage and that remains almost undamaged by the breakdown.I. INTRODUCTION
Many properties of electric thin-wire explosions (EWs) were first discovered during the initial period of extensive investigations in this field in the middle of the twentieth century. Spontaneous current breaks were observed during capacitor discharges through thin metal wires in air: the so-called current pause (or “dwell”).1 Such current breaks (either total or with the current decreasing to a negligible value of a few tens of amperes) in nanosecond discharges can continue for hundreds of nanoseconds, ended by secondary breakdown of the discharge gap, during which the current in the circuit is restored. (If the voltage across the electrode gap is insufficient for the breakdown of explosion products, an infinite current pause occurs.) The pause duration increases with increasing wire length and can vary in different ways with wire diameter. As the charging voltage is increased, the pause shortens until it disappears completely. The pause has been found to be dependent on the wire material: it is frequently observed for copper, silver, tin, and nickel wires, but never for tungsten.2 In the result, all metals were divided into two groups, depending on the presence or absence of current pauses in current oscillograms.3 The physical mechanism underlying this classification has been established as being based on a combination of the thermal and electrophysical properties of the metal: boiling temperature, heat of vaporization, and electric conductivity.4,5
The decrease in current observed during an EW is explained by a dramatic fall in the electrical conductivity of the metallic load material during fast resistive heating. This very short (on the discharge scale) stage of various vigorously proceeding phase transitions is in fact what constitutes the explosion of an electrical conductor. At this stage, in general, the metal experiences so-called phase and cavitation explosions, resulting in a dramatic increase in core volume and in the elimination of current flow in the core.6 The core represents the remains of the wire material and noticeably exceeds in density the surrounding corona. Inertial radial expansion of wire explosion products then occurs until complete disappearance of the wire. During the expansion stage, the current in the circuit can either cease for a long time (the current-pause EW regime) or continue to flow in the coronal plasma (the regime without a current pause).
The absence of a current pause for tungsten and other materials can be explained by efficient current shunting in the near-surface mixture of air, metal vapor, and desorbed impurities. The high emissivity of tungsten results from its high boiling temperature. This leads to early ionization and breakdown of the air surrounding the wire. The decrease in current in the core due to loss of conductivity is masked by the appearance of an additional highly conducting material—a coronal plasma. As a result, the current in the circuit is not interrupted and will decrease only slightly for some time (until its complete elimination from the core and the final establishment of a shunting channel).
The dynamics of the current distribution between the core and corona cannot be observed experimentally: electrotechnical measurements can give only the total current in the circuit. Visualization diagnostics is also non-informative here, because a wire with a typical initial diameter of the order of ten microns has no time to expand during this stage of the explosion. However, in Refs. 7 and 8, breakdown along a thin conductor surface was studied in the case of generation of shock waves during the explosion of a tungsten wire. Although there is little doubt regarding the decisive role of shunting in the EW process for wires made of tungsten or similar materials, many details of the complex mechanism of this breakdown remain unclear.
In the current-pause EW regime, the start of the explosive expansion and the moment of current resurge in the circuit are considerably separated in time. Obviously, the development of secondary breakdown is facilitated by a decrease in the density of the expanding material of the exploded load. However, the state of this material (vapor, drop–vapor mixture, or microparticles), its density, and its degree of homogeneity are not known reliably. The localization of the discharge channel itself also requires further investigation. In fact, secondary breakdown is a specific type of high-voltage discharge in a gas gap. The physics of gas discharges involves many unsolved problems, and therefore obtaining new experimental data in this field is of great importance. In this paper, the breakdown of the interelectrode gap at atmospheric pressure is studied for the secondary discharge in the explosion products of a thin copper wire—a material where a current pause can be readily achieved. The material becomes partially transparent in the visible region during expansion. This allows us to study the structure of explosion products during the final EW stage using laser photography of the discharge gap.
II. EXPERIMENTAL SETUP
Experiments were performed using a GVP generator based on a low-inductance capacitor with a storage capacitance of 0.1 μF and an inductance of 10 nH, which could be charged up to 35 kV. The circuit inductance was L = 340 nH and the electrode gap was l = 12 mm (Fig. 1). In the short-circuit mode, a sine-wave current pulse of amplitude Imax = 10 kA and rise time of about 430 ns was generated. Experiments were performed in air under normal conditions.
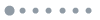
Figure 1.Experimental setup.
For fixed EW parameters (discharge-circuit inductance, wire load material, and geometry), the current pause duration in air is mainly determined by the applied voltage. (In fact, it is determined by the average strength of the longitudinal electric field E = U0/l. For microsecond EWs, accurate dependences of the current pause duration on the discharge parameters were obtained in Ref. 9.) In our experiments with a copper wire load 12 mm in length and 25 μm in diameter, the current pause disappeared when the charging voltage U0 was increased to 20 kV. Because of this, to work in the required regime, this voltage was set in the range 8–15 kV.
The voltage was measured with a resistive voltage divider made of low-inductance resistors; the current was measured with a 5-mΩ coaxial shunt, and the current derivative was measured with an inductive loop; this allowed us to determine the inductive component of the voltage on the divider. The resistive component of the voltage on the wire was determined as Ur = U − LdI/dt. Using these data, the energy deposited in the wire material, ε = ∫IUrdt, was calculated. Signals were detected with 500-MHz Tektronix TDS 3054B oscilloscopes.
The shadow images of the discharge channel loaded with a thin wire were obtained using 0.532-μm, 10-ns second-harmonic pulses from a Nd3+:YAG laser. A subsequently developed optical system allowed us to obtain three time-separated images for each laser shot. Delays between the channels could be varied from 15 to 50 ns. The time between the current start and a laser shot was controlled with a delay generator accurate to several nanoseconds. Imaging was performed with SIGMA objective lenses with focal distances 135–400 mm, and the images were recorded with Canon digital cameras. The spatial resolution of the images was ∼20 μm.
III. EXPERIMENTAL DATA AND DISCUSSION
The time dependence of the current during the electrical explosion of a thin copper wire has three characteristic regions: (1) the so-called “first current pulse”; (2) the complete break or noticeable current-decrease phase; and (3) secondary breakdown in the form of a decaying sinusoid—the final stage of the process [Fig. 2(a)]. In voltage waveforms, these regions correspond to an overvoltage peak, a constant-voltage “ledge” appearing at a level close to the charging voltage, and a sinusoidal “tail” [Fig. 2(b)]. If a current pause does not explicitly appear, the constant-voltage ledge is absent. These oscillograms clearly demonstrate that the applied voltage determines the main properties of the discharge. The lower the voltage, the smaller the front steepness and the maximum of the current amplitude and the later the secondary breakdown appears. These changes in the character of the waveforms are intuitively understandable. With increasing voltage, the deposited energy and therefore the rate of expansion of the explosion products increase. The density of the substance falls faster, so secondary breakdown occurs earlier. It should be noted here that a number of attempts have been made to simulate the process of copper wire explosion in a surrounding medium using various semiempirical numerical models.10–12 In all cases, the calculated current and voltage curves have been found to agree satisfactorily with experiment, including the current pause and the subsequent breakdown. However, the value of these approaches is limited by the broad use of fitting parameters, which is unavoidable due to the lack of clarity in the physical basis of the explosion process to date.
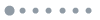
Figure 2.Examples of current (a) and voltage (b) oscilloscope signals of copper wire explosions at different initial voltages: (1) 20 kV (the energy deposited up to the moment of secondary breakdown is 17 J/mg); (2) 15 kV (9.7 J/mg); (3) 10 kV (7.7 J/mg).
It is important to note that the absence of a current pause for copper (for a high-charging-voltage EW) is not a sign of early shunting, in contrast to tungsten, for which it begins already at the melting stage. The rapid breakdown of the copper wire explosion products is facilitated by a considerable overvoltage across the electrodes caused by the “jump” in the impedance of the discharge gap at the moment of loss of metal conductivity. The overvoltage is more than twice the initial voltage and reaches ∼44 kV for U0 = 20 kV. For a 12-mm interelectrode gap, this voltage certainly provokes breakdown in air. On the other hand, for the “copper group” materials, strong expansion of the explosion products is typical and begins together with a dramatic voltage decrease.6 Poorly conducting material consisting mainly of a vapor–drop mixture fills the whole region behind the shock-wave front. This complicates shunting near the surface, and the discharge current is forced to flow for some time through the core. For more definite conclusions to be drawn, however, further investigation of this question is required; at present only qualitative reasoning is possible, based on an analysis of late optical images.
Figure 3(a) shows a typical image of copper wire explosion in air without a current pause. The vigorous nature of the processes occurring is obvious: different instabilities appear irregularly in the material and persist until the discharge ends. Their emergence can only be due to the discharge current. Consequently, its complete displacement from the products of the explosion (shunting) occurs here much later than in the case of tungsten. Such an EW scenario, which is sometimes called “internal,” has been considered previously in Refs. 6–8. The characteristic current and voltage waveforms for this explosion mode are shown in Fig. 3(b).
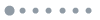
Figure 3.Shadow image (a) and oscilloscope signals (b) of copper wire explosion (wire diameter 25 μm, length 12 mm). U0 = 20 kV, without current pause.
An expanding wire looks significantly different during a current-pause EW [Fig. 4(a)]. In this case, the voltage across the interelectrode gap proves to be insufficient for rapid breakdown of the explosion products, which have a rather high resistance, and the current in the circuit ceases. In the absence of current, the core expansion occurs quietly without perturbations. The material appears to be uniformly distributed within the shock-wave front, except for small-scale regularly located transverse inhomogeneities (a stratified structure) and a characteristic “dark rim” 200–300 μm in thickness. The latter suggests the existence of density jump on the external boundary of the core and thus a tubular structure of this axially symmetric object. From this, we can assume that the transverse “strata” observed in the shadowgrams are not flat “layers,” as they are frequently represented, but rather are developing in the surface layer of a “tube,” as if they are modulating its wall thickness. The possibility of formation of a liquid-walled hollow core during the EW process under certain conditions has been reliably established (most probably, the core is filled with low-density material in the form of a foam or drop–vapor mixture). This has indeed been shown experimentally13–15 and by molecular simulations.16 A qualitative theoretical analysis of this process is presented in Ref. 17. Therefore, in our further interpretation of experimental results on the development of secondary breakdown in explosion products, we shall assume a tubular structure of the core.
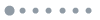
Figure 4.Shadow image (a) and scope signals (b) of copper wire explosion (wire diameter 25 μm, length 12 mm). U0 = 10 kV, with current pause.
Thus, the core material, which experiences no perturbations, continues to expand by inertia for a long time. (According to measurements of the core size in laser images at different moments during the discharge, the expansion rate for U0 = 10 kV at the beginning of the current pause is 2.1 ± 0.03 μm/ns and decreases by almost half by its end.) The discharge circuit remains open all this time, and a voltage close to the charging voltage continues to be applied across the interelectrode gap [Fig. 4(b)]. The material density inside the “tube” gradually decreases during expansion, and conditions for a breakdown appear there at a certain moment. Laser probing visualizing the internal structure of the core demonstrates the traces of the breakdown current flow as darkened regions appearing owing to variations in the local index of refraction caused by changes in plasma parameters produced by the current (see, e.g., the breakdown channel in Fig. 5).
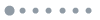
Figure 5.Shadow image of copper wire explosion (wire diameter 25 μm, length 12 mm) at 135 ns after beginning of secondary breakdown. U0 = 10 kV, linear breakdown channel.
Analysis of the images obtained showed that in most cases the secondary breakdown can be assigned to one of the three characteristic types (for all charging voltages used in the experiments): linear, spiral, and axial.
In shadowgrams, linear breakdown looks like a straight dark line connecting the electrodes (Fig. 5). Sometimes this breakdown is supplemented with weaker and shorter channels close to the main channel. The main breakdown goes along the internal wall of the tubular core, in its upper or lower part (in the probing projection). Such an asymmetry can be related, as a rule, to the initial location of the wire. Fixing a 25-μm wire strictly at the center of semicircular horizontally arranged electrodes with the help of standard clamping screws was by no means always possible. On both the cathode and anode, the vertical displacement could be as much as 1–2 mm (because of this, the wire was sometimes placed at a small angle to the horizontal axis). In all such cases, the radial expansion of explosion products symmetric with respect to the initial wire proved to be nonsymmetric with respect to the discharge gap axis (Fig. 5). The most natural breakdown path is along the internal surface of the “tube,” at the density jump boundary, while the choice of the azimuth in each shot is determined by the closeness of the breakdown line to the electrode axis—the shortest distance between electrodes, where the longitudinal field strength is maximal. If the wire was pulled above the axis, as in Fig. 5, the breakdown usually developed in the lower part of the core, and vice versa. Note that linear breakdown dominated in our experiments.
Spiral (or branching) breakdown, which is much less common, looks like a randomly bent tube connecting the electrodes (Fig. 6). Visually, it occupies the total volume of the explosion products and sometimes is separated into “sleeves.” It is difficult to reconstruct the real shape of a shadow image just from its projection. However, one can clearly see that the stratified structure is destroyed only where the breakdown emerges on the external surface of the core. This suggests that the discharge predominantly developed along the internal surface of the tubular core, not interacting with strata in its wall. The winding trajectory of the breakdown resembles the well-known shape of lightning channels and is probably related to random inhomogeneities of material on the internal surface of the core. It seems that a breakdown of this type was observed in Ref. 13, where photographs of copper wire explosions inside a tubular core also exhibit spiral discharge channels.
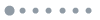
Figure 6.Shadow image of copper wire explosion (wire diameter 25 μm, length 12 mm) at 80 and 100 ns after beginning of secondary breakdown. U0 = 15 kV, spiral breakdown channel.
The axial breakdown type looks in shadowgrams like an expanding cylinder (Figs. 7 and 8). Except for near-electrode regions, the cylinder is virtually straight and obviously hollow, the features of the core structure being clearly seen through it. The symmetric type of expansion suggests that in this case the discharge developed not near the internal surface of the core, but on its axis. This is confirmed by the complete absence of any distortions of the stratified structure. A time sweep (three successive frames obtained in one shot) shows that the cylindrical channel expanded at a rate of 4.5 μm/ns, which is twice the rate for the core as a whole. Note that it is likely that we see in shadowgrams not the direct trace of the current (as in the linear type of breakdown), but rather the front of a cylindrical shock wave symmetrically diverging around the axial discharge. It is doubtful that the current can stably exist in the form of a tube with such a large diameter, unlike a shock wave propagating in a transparent medium. The absence of the current trace itself suggests that the density of the residual material inside the core is low for this type of secondary discharge. [We can assume that this is also the case for spiral breakdown, with a correction for the winding trajectory of the current producing a shock wave (Fig. 6). Unfortunately, all the images of this type of breakdown were obtained in a single-frame photographic regime, and therefore it was impossible to observe the assumed cylindrical expansion of the channel.] In any case, shadowgrams of shock waves in electrical discharges in open air look similar (see, e.g., the shadow images of air-gap breakdown in Ref. 18).
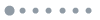
Figure 7.Shadow image of copper wire explosion (wire diameter 25 μm, length 12 mm) at 175, 205, and 245 ns after beginning of secondary breakdown. U0 = 10 kV, axial breakdown channel. All three images were obtained in one shot.
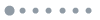
Figure 8.Shadow image of copper wire explosion (wire diameter 25 μm, length 12 mm) at moments 25 ns before and 5 ns and 45 ns after beginning of secondary breakdown. U0 = 10 kV, axial breakdown channel. All three images were obtained in one shot.
One of the shots in this series demonstrates the moment of breakdown generation on the core axis (Fig. 8). In the first of three frames, during the current pause, material remains unperturbed; in the second frame, obtained 5 ns after the beginning of secondary breakdown, a trace of width 130 μm appears on the axis, which transforms in the next frame, after 40 ns, to a transparent tube. The diameter of this tube is noticeably smaller than for the shot in Fig. 7, because a shorter time elapsed after the onset of breakdown. The expansion rate of the cylindrical channel observed immediately after the onset of breakdown was about 7 μm/ns.
Depending on the properties of the medium in which a breakdown is studied, two alternative scenarios are possible. (1) The density of the residual matter inside the core, which initially (at the start of the current pause) is relatively high, decreases during expansion, and achieves the Paschen curve minimum first on the axis. (2) In contrast, the core is initially almost hollow; its wall material gradually evaporates and, owing to symmetry, is collected on the axis and then serves as a seed for the secondary breakdown current. At present, there is insufficient experimental information to allow a choice between these scenarios. Note, however, that this and the other breakdown types described above are incompatible with the popular concept of the core as an object homogeneous in the radial direction and separated along the axis by flat strata. Therefore, the hypothesis in Refs. 3 and 19 that the secondary discharge results from successive connections of neighboring flat “disks” by local arcs seems unlikely.
Experimental data on the existence of different (two) types of secondary breakdown have previously been presented in the literature only for microsecond EWs. A study of the expansion of copper and constantan wires in air showed that as the applied voltage was increased from 6 to 20 kV, the breakdown type changed with high probability.20 Primarily, this is related to the position of discharge initiation. At lower voltages, the breakdown was initiated on the wire surface, but for higher voltages, initiation occurred inside the wire. Here, the external breakdown always involved a short current pause, whereas for the internal breakdown that appeared above a certain critical voltage, a considerably longer current pause was observed. In principle, for any voltage exceeding the threshold, either of these two discharge types (with a longer or shorter current pause) could be initiated, but with noticeably different probabilities. Thus, the current pause is not always a unique function of voltage, being considerably dependent on the breakdown type.
The specific nature of the physical processes in nanosecond EWs means that we cannot compare our results directly with the data presented in Ref. 20, where, in addition, quite different diagnostics were used (photography of the discharge gap using a frame optical streak camera). Nevertheless, the internal breakdown described in Ref. 20 is very similar to the axial breakdown in our experiments. This is a contracted current cord, spirally twisted near the electrodes, that appears on the wire axis at the end of the current pause and then rapidly expands (cf. Fig. 7 or 8). In addition, in our experiments, we also found a dependence of the current pause duration on discharge type, although it was different, as we will show below.
As a rule, in real experiments, it is impossible to reproduce absolutely exactly the parameters that are important for initiating the secondary discharge: the wire length and the homogeneity of its material, including its surface state. In addition, the charging voltage in our experiments was accurate only to ∼10%. As a consequence, there is some spread in the onset time of secondary breakdown. From this point of view, the energy deposited into matter during the first current pulse is a more appropriate parameter compared with the nominal applied voltage and geometrical parameters. The energy deposition automatically takes into account many factors that are important for discharge dynamics but are poorly controlled in experiments. Figure 9(a) presents current waveforms obtained during copper wire explosions for the same charging voltage U0 = 15 kV, but with slightly different energy depositions in different shots, varying between 0.47 and 0.6 J. We found that the current pause duration increased with decreasing energy deposition, as expected. In particular, the current pause “jumped” to a higher duration corresponding to the minimum energy deposition. It was more surprising that this monotonic dependence also held for the whole group of closed waveforms. This suggests that, rather than a statistical spread in the breakdown onset time, there is a quite clear regularity, more accurate than a simple voltage dependence.
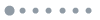
Figure 9.(a) Current oscilloscope signals in shots with linear breakdowns and (b) current pause durations versus deposited energies for shots with different breakdown types at an initial voltage of 15 kV (copper wire of diameter 25 μm).
However, this dependence is satisfied well only for shots in which the secondary breakdown is linear [upper blue dots in Fig. 9(b)], and is not inherent to discharges of other types. For example, Fig. 10 shows current and voltage waveforms obtained in the same experimental series under identical conditions. In three shots with current pauses close in duration (the 5th, 8th, and 9th shots), linear secondary breakdown was detected. For these, as for the shots in Fig. 9, the “normal” dependence of the energy deposition can be seen: the higher the energy deposition, the shorter the current pause. Note that in this case the voltage at breakdown dramatically decreased, indicating the high conductivity of the medium in the discharge channel.
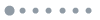
Figure 10.Current (a) and voltage (b) oscilloscope signals obtained under identical experimental conditions (15 kV, copper wire of diameter 25 μm).
Two other waveforms (the 6th and 7th shots) with short current pause correspond to spiral breakdown. In this case, the energy deposition shows the opposite dependence. The discharge current was lower and the voltage decrease was rather smooth. This means that the discharge channel resistance and/or inductance at breakdown was higher than in the previous case. We can assume that, for some reason, the ionization of the medium was more efficient here, and the required number of current carriers was formed until the core had time to expand enough for decreasing its density down to the “breakdown” value. The discharge began to develop under unfavorable conditions of a dense medium and was forced to “choose” regions with minimum local resistance. As a result, the trajectory became winding and the total length of the discharge channel increased. Because of this, such breakdowns, which start earlier, prove to be longer than linear secondary breakdowns.
The red current and voltage curves (4th shot) correspond to discharges of axial type, with a cylindrical breakdown channel. In terms of current pause duration, this is an intermediate case, while, with regard to energy deposition, such discharges do not exhibit any of the dependences described above.
Figure 10(b) clearly shows that the “ledge” height (i.e., the voltage in the current pause at which secondary breakdown begins) coincides for all shots and therefore for all breakdown types. At the same time, the amplitudes of the corresponding currents in Fig. 10(a) noticeably differ. This means at least that the impedances of the discharge gap are different for breakdowns of different types. At present, it is difficult to explain this difference, because the material in which the breakdown developed was, in all cases, formed during the EW for very similar discharge parameters.
Of course, the onset of any gas discharge is, by its nature, random to some extent. It requires both that there be a sufficient number of current carriers and that the medium itself, which is not necessarily homogeneous, be ready for discharge to occur. The development of breakdown can be affected not only by the structure of the core and the state of its material, but also by a number of other factors that have been neglected so far. To clarify and interpret this complicated picture, further investigations are required.
IV. CONCLUSIONS
We have studied nanosecond electric explosions of thin copper wires in air in the current-pause regime at voltages of 10–15 kV (in the energy deposition range 9–14 J/mg). Analysis of shadow images of the discharge gap obtained at different times has shown that the explosion products under these conditions form a stable tubular structure. This structure remains almost unchanged for a few hundreds of nanoseconds as the core continues to expand by inertia. The system of strata, which we believe to belong to the external shell of the core, remains undisturbed, both during the current pause and at the secondary breakdown stage during current regeneration in the circuit. In addition, new volume perturbations, which usually accompany high-current discharges in matter, do not appear. The images of secondary discharge channels have allowed us to interpret this discharge as being internal with respect to the tubular core. The discharge current can flow either along the axis of the hollow core or near its internal surface. The breakdown on the external surface of the wire (core) reported in Ref. 20 has not been observed in our experiments.
We have found that at the same charging voltage (and the same energy deposition), three types of secondary breakdown can appear, differing in current-pause duration and, in a certain sense, in symmetry type. The longest current pause occurs for linear breakdown and the shortest for circular (twisted) breakdown. Axial breakdown (with the formation of a cylindrical shock wave) is an intermediate type and exhibits a current-pause duration that is also intermediate in value. The usual inverse dependence of current-pause duration on energy deposition is valid only for the linear discharge type.
References
[1] J. Wrana. Arch. Elektrotech., 33, 656(1939).
[2] N. N. Sobolev. J. Expt. Theor. Phys. USSR, 17, 986(1947).
[3] I. F. Kvartskhava, A. A. Chernov, A. A. Pliutto, V. V. Bondarenko. Sov. Phys. JEPT, 8, 40-51(1956).
[4] H. Hilton, H. F. Webb, P. H. Levine, W. G. Chace, A. V. Tollestrup, H. K. Moore. Exploding Wires, 37-75(1962).
[5] S. M. Karakhanov. Sov. Phys. Tech. Phys., 23, 832(1978).
[6] S. A. Pikuz, A. R. Mingaleev, A. E. Ter-Oganesyan, T. A. Shelkovenko, G. V. Ivanenkov, V. M. Romanova. Plasma Phys. Rep., 41, 617-636(2015).
[7] S. A. Pikuz, D. V. Barishpoltsev, T. A. Shelkovenko, A. R. Mingaleev, V. M. Romanova, A. E. Ter-Oganesyan, G. V. Ivanenkov, S. I. Tkachenko. Phys. Plasmas, 14, 123502(2007).
[8] D. V. Barishpol’tsev, S. Yu. Gus’kov, G. V. Ivanenkov. Plasma Phys. Rep., 36, 67-76(2010).
[9] A. Vlastos. J. Appl. Phys., 38, 4993-4998(1967).
[10] G. R. Prieto, L. Bilbao. Appl. Sci., 7, 829(2017).
[11] X. Li, G. Yin, S. Jia. IEEE Trans. Plasma Sci., 46, 972(2018).
[12] Y. S. Hwang, K.-J. Chung, K. Lee, D.-K. Kim. J. Appl. Phys., 120, 203301(2016).
[13] W. Muller, W. G. Chace, H. K. Moore. Exploding Wires, 186-208(1959).
[14] W. G. Chace, T. Korneff. Rev. Sci. Instrum., 42, 1184(1971).
[15] T. A. Shelkovenko, C. L. Hoyt, S. A. Pikuz, A. R. Mingaleev, V. M. Romanova, I. N. Tilikin, D. A. Hammer, J. D. Douglass. IEEE Trans. Plasma Sci., 43, 2520-2526(2015).
[16] S. A. Pikuz, V. V. Zhakhovsky, P. F. Knapp, P. V. Sasorov, T. A. Shelkovenko, S. I. Tkachenko, Ch. C. Saylor, D. A. Hammer. AIP Conf. Proc., 1426, 1207(2012).
[17] A. R. Mingaleev, S. A. Pikuz, G. V. Ivanenkov, I. N. Tilikin, T. A. Shelkovenko, V. M. Romanova, A. E. Ter-Oganesyan. Phys. Plasmas, 25, 112704(2018).
[18] V. M. Romanova, A. V. Agafonov, S. I. Tkachenko, E. V. Parkevich, T. A. Shelkovenko, S. A. Pikuz, A. R. Mingaleev. J. Expt. Theor. Phys., 124, 531-539(2017).
[19] A. I. Savvatimskii, S. V. Lebedev. High Temperature, 494-500(1970).
[20] A. Vlastos. J. Appl. Phys., 39, 3081-3087(1968).