Abstract
A coherent combination of emission power from an array of coupled semiconductor lasers operating on the same chip is of fundamental and technological importance. In general, the nonlinear competition among the array supermodes can entail incoherence and spectral broadening, leading to a spatiotemporally unstable and multimode emission pattern and thus poor beam quality. Here, by harnessing notions from supersymmetric (SUSY) quantum mechanics, we report that the strategic coupling between a class III-V semiconductor microring laser array with its dissipative superpartner can be used to limit the number of supermodes available for laser actions to one. We introduce a novel approach based on second-order SUSY transformation in order to drastically simplify the superpartner array engineering. Compared to a conventional laser array, which has a multimode spectrum, a SUSY laser array is observed to be capable of operating in a single (transverse) supermode. Enhancement of the peak output intensity of the SUSY laser array has been demonstrated with high efficiency and lower lasing threshold, compared with a single laser and a conventional laser array. Our experimental findings pave the way towards broad-area and high-power light generation in a scalable and stable fashion.1. INTRODUCTION
Supersymmetric (SUSY) quantum field theory predicts that each elementary particle and antiparticle of the standard model has its own partner—a superpartner (or sparticle)—endowed with opposite statistics [1,2]. A space–time symmetry transformation between a particle and its superpartner theoretically resolves the puzzle of the Higgs mass renormalization (the hierarchy problem) in particle physics beyond the standard model; the loop contributions of one particle to the Higgs field are exactly cancelled by the contributions of its superpartner, thus confirming the observed mass [3]. While the search for sparticles is being continued, the ramification of the SUSY theory has attracted attention in other branches of physics [4]. In optics, for example, the isomorphism between the Schrödinger equation and the scalar Helmholtz equation facilitates utilizing the mathematics of SUSY for the strategic manipulation of light scattering, guiding, and localization in novel photonic materials and structures. In the framework of unbroken SUSY, starting from a given primary optical structure, it is possible to construct its superpartner with an identical eigen-spectrum apart from the fundamental mode, which is deleted by the SUSY transformation [5–7]. When the former structure is judiciously coupled with its unbroken superpartner, the global mode matching of the combined structure leverages efficient mode conversion and multiplexing in passive configurations [8], and strategic selection of a single transverse supermode in an active laser array [9,10].
Integrated semiconductor laser arrays that consist of a large number of densely packed emitters are of special importance for the next generation of applications such as material processing, broad-area displays, industrial heating, and lidars [11–13]. Coherent combination and phase locking are essential in obtaining higher power output from a large transverse area than that available from a single semiconductor laser. This has offered advantages of narrowing the spatial extent of the radiation beam while maintaining a narrower output spectrum [14–18]. However, a laser array, in general, operates on many closely spaced transverse modes (supermodes) of the same longitudinal order; the degeneracy of each cavity longitudinal mode is lifted owing to the proximity coupling among the individual cavities [Fig. 1(a)]. Nonlinear modal competition due to limited gain often results in pulsation and filamentation that degrade the spectral, spatial, and temporal characteristics of the radiation [19,20]. While the single longitudinal mode lasing was addressed by parity-time symmetry [21], novel strategy and symmetry consideration are required to support single transverse supermode lasing. Sophisticated laser-array designs based on gain tailoring, special diffraction, or non-Hermitian coupling are proposed to mitigate the complexity of transverse supermode lasing [22–24].
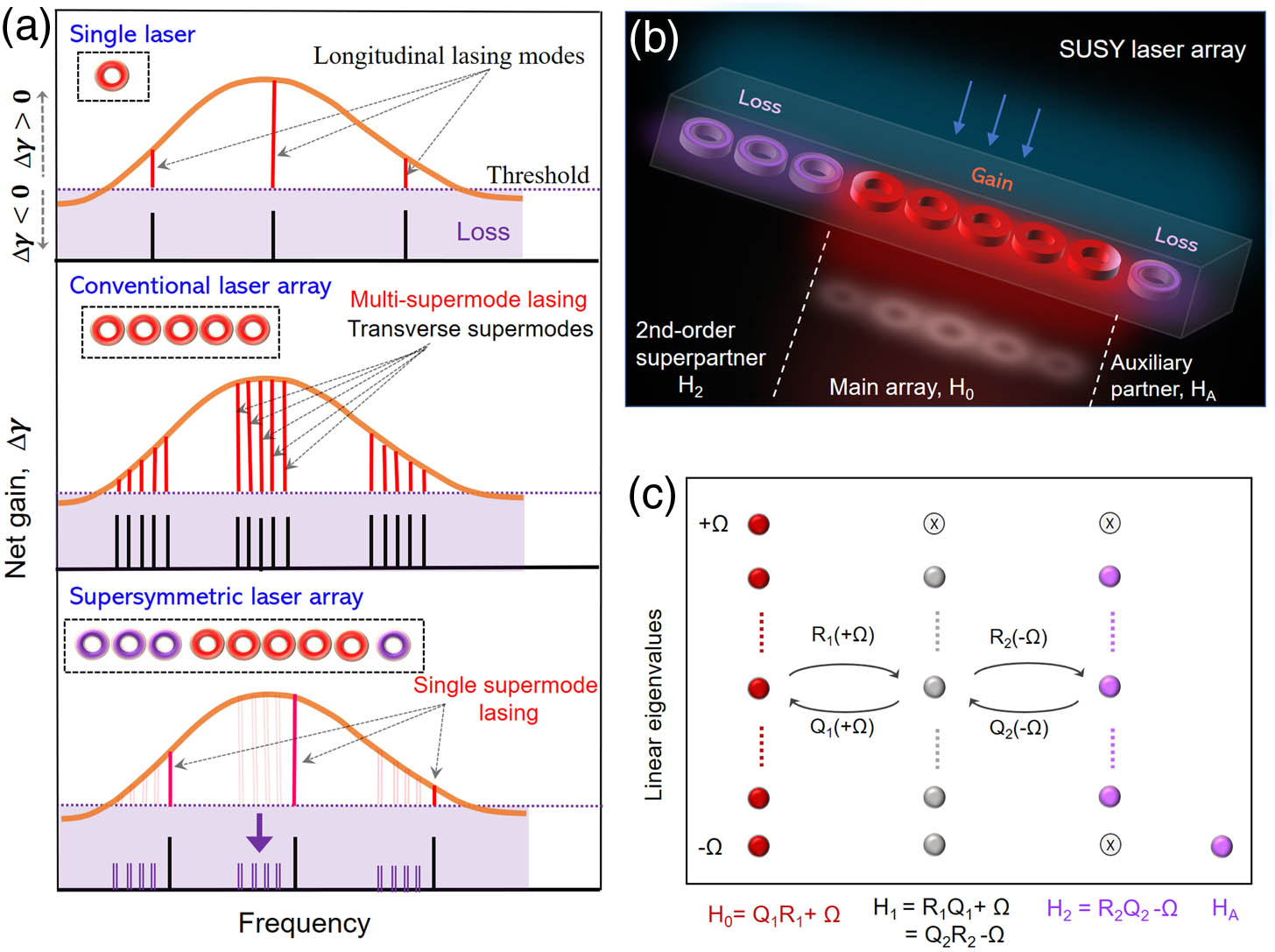
Figure 1.Fundamental concept behind the single (transverse) supermode lasing. (a) A single laser supports multiple longitudinal cavity modes (vertical black lines) separated by free spectral range. When such rings are coupled, closely spaced transverse supermodes emerge with equal threshold (middle panel). In the presence of gain, a suitable pump can induce simultaneous lasing of all the supermodes. The global mode coupling with a dissipative superpartner in a SUSY laser array, schematically shown in (b), can increase the threshold of undesired modes (shown in vertical purple lines in the bottom panel), enforcing a lasing of a single supermode that lacks a superpartner counterpart. (c) Second-order SUSY transformation for the superpartner design.
In this paper, we theoretically analyze and experimentally demonstrate single (transverse) supermode lasing in a SUSY designed array [schematically shown in Fig. 1(b)] of III-V semiconductor active microring resonators. With this aim, we construct a superpartner array whose linear spectrum is identical to that of the main array apart from the fundamental supermode. The operating principle of the single-supermode SUSY laser array then follows from the judicious coupling of the main array with its dissipative superpartner, which ensures the reduction of supermode competition by decreasing the number of available lasing modes [9]. The global mode matching between the SUSY partners ensures the suppression of all but the fundamental supermodes, whose modal loss and thus lasing threshold are increased, owing to the added stronger loss in the superpartner channels [Fig. 1(a)]. The fundamental supermode, confined only in the main array lacking a superpartner, has the lowest threshold and is favorable for lasing upon suitably applied pumping. The advantage of the fundamental supermode selection is that the emissions from all the rings are in phase. Although a laser is a highly nonlinear system, the SUSY linear-mode suppression mechanism mentioned above is observed to persist in our experiment. Compared to a conventional laser array, which is highly multimoded, our SUSY array is demonstrated to support a single supermode lasing corresponding to two nearby longitudinal orders. Apart from its single-mode property, the SUSY laser array is observed to be highly efficient, requiring a lower lasing threshold, and has enhanced intensity compared to a single laser and a conventional laser array at the identical pumping condition.
Sign up for Photonics Research TOC. Get the latest issue of Photonics Research delivered right to you!Sign up now
2. THEORY
While a superpartner resulting from a single SUSY transformation (deletion of a single eigenmode) was proposed for a single-mode SUSY laser array in theory [9], the resulting superpartner elements have small but non-negligible frequency detuning, which can be detrimental, especially if the coupling between the lasers is much weaker compared to the onsite energies (as is the case of microring lasers) [10]. Here the challenge is overcome by the introduction of a novel design technique based on two consecutive SUSY transformations applied to a homogeneous array enabling elimination of two supermodes with the largest and smallest frequencies, respectively [Fig. 1(c)]. The second-order superpartner array thus obtained consists of identical elements with the resonance frequency identical to that of a main array element.
We consider a homogeneously coupled one-dimensional array of identical laser cavities with the resonance frequency (considered to be the lowest transverse order), nearest-neighbor coupling strength , cavity linear loss , and nonlinear gain . In the absence of gain, the linear modes of the system are given by , where is a tridiagonal matrix with diagonal entries , off-diagonal entries , and , , are the eigenfrequencies. Note that the normalized eigenvalues appear to be symmetric with respect to zero, such that . The first-order superpartner of is obtained by the discrete SUSY transformation (QR-factorization algorithm [10]) , , which implies that the fundamental frequency is deleted from the otherwise isospectral spectrum of , i.e., . A repeated application of the similar procedure at the frequency yields a second-order superpartner , such that and . In this case, , and the linear eigenmodes of and are related by and . This implies that a first-order superpartner is spectrally equivalent to a pair of subarrays (, ) containing the second-order superpartner and an isolated auxiliary partner (which consists of a single element of frequency ). The coupling of the main array with the lossy subarrays and [as shown in Figs. 1(b) and 2(a)] then ensures energy dissipation of all the isospectral supermodes, while the fundamental supermode remains unaffected provided that the total coupling between the arrays is much weaker than . The SUSY laser array, so constructed, thus consists of lasers— belongs to the main array, to , and a single laser belong to the . The most remarkable property of is that all of its elements have zero relative frequency detuning, while the couplings between the elements remain inhomogeneous. The second-order SUSY technique thus drastically simplifies the large-scale laser array engineering, as all the laser elements but the auxiliary one are identical in geometry.
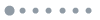
Figure 2.SUSY laser array linear mode analysis. (a) Design parameter of a SUSY microring laser array containing nine coupled lasers. (b), (c) Linear eigen spectrum and corresponding modal intensities (only the cross-sectional view is shown), respectively. The spectrum shows that the fundamental mode of the SUSY array has the least threshold. All other modes of the conventional laser array split into symmetric and anti-symmetric pairs . Here , , , and are considered.
To experimentally validate and compare the spectral responses of the above-mentioned SUSY laser array, we have designed a single microring laser, a conventional array of five lasers (without superpartner), and the SUSY array of nine lasers. The design parameters and the corresponding linear eigenmode analysis are shown in Fig. 2. The cavity intrinsic loss is considered equal for all the rings (in the main as well as superpartner array), while additional loss is introduced in the superpartner lasers. To avoid mode decoupling between the main and superpartner subarrays, the loss contrast must be less than the coupling between the subarrays. As seen in Fig. 2(b), the conventional laser array has five eigenfrequencies, each with an equal amount of loss, whereas the SUSY laser array possesses enhanced losses to all the modes except for the largest frequency. Finite element simulations were performed to obtain the corresponding transverse-electric polarized field intensity distributions in the InGaAsP laser array in a silica substrate. The additional loss is estimated by considering a thin layer of Cr/Ge on the ring structures, and the coupling between the lasers is tailored by adjusting the center-to-center distance between the rings. The results are shown in Fig. 2(c). Apart from the fundamental supermode that is predominantly confined in the main array, other supermodes have significant overlap with the lossy superpartner subarrays, leading to the single transverse mode lasing.
3. EXPERIMENT
The devices were fabricated by electron-beam lithography and plasma etching on 200 nm thick InGaAsP multiple quantum wells as the gain material, with the gain bandwidth spanning from 1430 nm to 1600 nm. The scanning-electron-microscope images of the SUSY array sample with the crucial geometric parameters are shown in Fig. 3(a). To ensure the loss required in the superpartner array, and to properly match the frequency detuning in the auxiliary ring, thin layers of Cr/Ge were appropriately deposited on the corresponding rings through aligned overlay electron beam lithography and lift-off. After using plasma-enhanced chemical vapor deposition to deposit a 3 μm thick layer of for protecting the sample, the InGaAsP ring lasers on the InP wafer were then bonded with a glass slide, followed by selective wet etching using to remove only the InP substrate. Each ring was 4 μm in outer radius, 500 nm in width, and 200 nm in height. Coupling between the rings in the main array, placed 150 nm distance apart, was experimentally measured to be 105 GHz around 1550 nm wavelength. The gaps between the rings in were 200 nm and 225 nm, while the gaps between the main and partner subarrays were 280 nm.
![Device and spectrum. (a) Scanning electron microscope images of the fabricated SUSY microring laser array. The image was taken before the transfer of the sample into a silica substrate. (b) Evolution of the photon emission spectrum from photoluminescence to amplified spontaneous emission and then to supermode lasing at the wavelengths of about 1544 nm and 1568 nm, when pumping is increased. The two lasing peaks, separated by 24 nm, belong to two longitudinal modes in a single microring laser [see Fig. 4(a)].](/Images/icon/loading.gif)
Figure 3.Device and spectrum. (a) Scanning electron microscope images of the fabricated SUSY microring laser array. The image was taken before the transfer of the sample into a silica substrate. (b) Evolution of the photon emission spectrum from photoluminescence to amplified spontaneous emission and then to supermode lasing at the wavelengths of about 1544 nm and 1568 nm, when pumping is increased. The two lasing peaks, separated by 24 nm, belong to two longitudinal modes in a single microring laser [see Fig. 4(a)].
The single rings and the ring arrays were pumped optically by a nanosecond pulsed laser with a 50 kHz repetition rate and an 8 ns pulse duration at the wavelength of 1064 nm. To uniformly pump each elementary ring in the ring array, the transverse profile of the pumping laser beam was shaped in a linear manner by a cylindrical lens and a near-infrared (NIR) objective with 0.4 numerical aperture. The emissions of light oscillating in the laser array were collected by another NIR long-working-distance objective whose spectra were subsequently measured by a monochromator. The emission spectrum of a SUSY array is shown in Fig. 3(b). Two lasing peaks around 1544 nm and 1568 nm wavelengths emerge when pump densities are gradually increased. The comparison of the emission spectrum with that of a single element laser and a conventional five-ring array under the same pump intensity is shown in Fig. 4(a). On one hand, the spectrum of the SUSY arrangement appears almost indistinguishable from the single ring narrow spectrum, implying that the SUSY ring supports single-supermode lasing. On the other hand, the spectrum of a conventional five-ring array, as expected, shows multiple peaks, which reflects the mode competition between the supermodes. The imaged lasing emission profiles from each of these cases are shown in the inset in Fig. 4(a). From the image of the SUSY array, we observe the light radiation only from the five main rings; the presence of the superpartner rings serves only to suppress an unwanted mode. Although the conventional laser array possesses a total emission power comparable to the SUSY array, the peak intensity produced by this SUSY laser is significantly enhanced owing to the single supermode nature. The characteristic light–light curves for a single element laser and the SUSY laser array are shown in Fig. 4(b). The lasing intensity produced by this SUSY laser is over four times higher than that from the single elementary laser at the pump density. The much steeper slope implies that the SUSY array is highly efficient compared to a single ring, indicating that the large transverse gain in the former case increases overall efficiency.
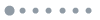
Figure 4.Comparison of measured spectra. (a) Output spectra for a single laser, a conventional laser array, and the SUSY laser array when pump intensity is considered. The conventional array is seen to be highly multimoded (as evident from the magnified spectrum shown in the inset). The spectra of a single laser and the SUSY laser array are almost indistinguishable. The enhancement of lasing output peak intensity is seen in the SUSY laser array. The nearfield images of lasing emissions are also shown. (b) Light–light curve showing the lowering of the threshold and enhancement of lasing output in a SUSY laser array compared to a single microring laser.
4. CONCLUSION
We report the first experimental observation of highly efficient, low-threshold, broad-area light generation from a microring laser array designed according to the principle of unbroken SUSY. The laser array is demonstrated to operate predominantly in a single transverse supermode of two adjacent longitudinal orders. The elegant strategy of dissipative coupling between the SUSY partner arrays is validated to suppress undesired modes in favor of fundamental mode lasing, where all individual laser cavities oscillate in phase. The notion of a second-order superpartner introduced here is conducive for large-scale SUSY laser array design. Our experimental results open the door for high-power light sources. It would be worthwhile to explore the interplay between parity-time symmetry and SUSY in a large-scale laser array to tailor the high-power light emission restricted to a single longitudinal as well as single transverse mode for ultimate spectral purity.
Note added. The preprint [25] reported a similar experiment on an active waveguide array.
Acknowledgment
Acknowledgment. This work was carried out in part at the Singh Center for Nanotechnology, part of the National Nanotechnology Coordinated Infrastructure Program, which is supported by the National Science Foundation grant NNCI-1542153.
References
[1] M. Drees, R. Godbole, P. Roy. Theory and Phenomenology of Sparticles(2004).
[2] M. Dine. Supersymmetry and String Theory: Beyond the Standard Model(2007).
[3] S. Dimopoulos, S. Raby, F. Wilczek. Supersymmetry and the scale of unification. Phys. Rev. D, 24, 1681-1683(1981).
[4] G. Junker. Supersymmetric Methods in Quantum and Statistical Physics(1996).
[5] S. Longhi. Invisibility in non-Hermitian tight-binding lattices. Phys. Rev. A, 82, 032111(2010).
[6] M.-A. Miri, M. Heinrich, R. El-Ganainy, D. N. Christodoulides. Supersymmetric optical structures. Phys. Rev. Lett., 110, 233902(2013).
[7] B. Midya, W. Walasik, N. M. Litchinitser, L. Feng. Supercharge optical arrays. Opt. Lett., 43, 4927-4930(2018).
[8] M. Heinrich, M.-A. Miri, S. Stützer, R. El-Ganainy, S. Nolte, A. Szameit, D. N. Christodoulides. Supersymmetric mode converters. Nat. Commun., 5, 3698(2014).
[9] R. El-Ganainy, L. Ge, M. Khajavikhan, D. N. Christodoulides. Supersymmetric laser arrays. Phys. Rev. A, 92, 033818(2015).
[10] W. Walasik, B. Midya, L. Feng, N. M. Litchinitser. Supersymmetry-guided method for mode selection and optimization in coupled systems. Opt. Lett., 43, 3758-3761(2018).
[11] D. Botez, D. R. Scifres. Diode Laser Arrays(1994).
[12] T. Y. Fan. Laser beam combining for high-power, high-radiance sources. IEEE J. Sel. Top. Quantum Electron., 11, 567-577(2005).
[13] M. C. Soriano, J. García-Ojalvo, C. R. Mirasso, I. Fischer. Complex photonics: dynamics and applications of delay-coupled semiconductors lasers. Rev. Mod. Phys., 85, 421-470(2013).
[14] D. R. Scifres, R. D. Burnham, W. Streifer. Phase-locked semiconductor laser array. Appl. Phys. Lett., 33, 1015-1017(1978).
[15] D. Scifres, W. Streifer, R. Burnham. Experimental and analytic studies of coupled multiple stripe diode lasers. IEEE J. Quantum Electron., 15, 917-922(1979).
[16] E. M. Philipp-Rutz. Spatially coherent radiation from an array of GaAs lasers. Appl. Phys. Lett., 26, 475-477(1975).
[17] A. F. Glova. Phase locking of optically coupled lasers. Quantum Electron., 33, 283-306(2003).
[18] E. Kapon, J. Katz, A. Yariv. Supermode analysis of phase-locked arrays of semiconductor lasers. Opt. Lett., 9, 125-127(1984).
[19] J. Ohtsubo. Semiconductor Lasers: Stability, Instability and Chaos(2013).
[20] H. G. Winful, S. S. Wang. Stability of phase locking in coupled semiconductor laser arrays. Appl. Phys. Lett., 53, 1894-1896(1988).
[21] L. Feng, Z. J. Wong, R. M. Ma, Y. Wang, X. Zhang. Single-mode laser by parity-time symmetry breaking. Science, 346, 972-975(2014).
[22] J. Katz, S. Margalit, A. Yariv. Diffraction coupled phase-locked semiconductor laser array. Appl. Phys. Lett., 42, 554-556(1983).
[23] D. Brunner, I. Fischer. Reconfigurable semiconductor laser networks based on diffractive coupling. Opt. Lett., 40, 3854-3857(2015).
[24] S. Longhi, L. Feng. Mitigation of dynamical instabilities in laser arrays via non-Hermitian coupling. APL Photon., 3, 060802(2018).
[25] M. P. Hokmabadi, N. S. Nye, R. El-Ganainy, D. N. Christodoulides, M. Khajavikhan. Supersymmetric laser arrays(2018).