
- High Power Laser Science and Engineering
- Vol. 8, Issue 2, 02000e20 (2020)
Abstract
1 Introduction
The next generation of laser facilities will offer high-energy pulses as well as high repetition rates, enabling ‘real-world’, high-throughput applications and a wide range of science that requires the acquisition of high volumes of data. Such applications include advanced materials processing and laser shock peening[
The Center for Advanced Laser Technology and Applications (CALTA) at STFC’s Central Laser Facility (CLF) is a pioneer of ‘DiPOLE’ multi-slab, cryogenic gas cooled Yb:YAG amplifier technology. In 2015, a single-stage prototype cryogenic gas cooled amplifier, called the DiPOLE system, achieved an output in excess of 10 J at 10 Hz, with an optical-to-optical efficiency of 22%[
In this paper, we report the scaling up of DiPOLE technology to a pulse energy of 150 J at 1 Hz. To the best of our knowledge, this is the highest energy achieved by a diode-pumped solid-state laser. These results were obtained during the development of a two-stage cryogenic gas cooled amplifier, called D100X, to be supplied to the European XFEL facility[
Sign up for High Power Laser Science and Engineering TOC. Get the latest issue of High Power Laser Science and Engineering delivered right to you!Sign up now
2 Setup and results
Figure
As precise control over the temporal profile is important for achieving efficient densification of materials in HED experiments, an automated pulse shaping capability was incorporated into the D100X laser system. A typical example is shown in Figure
To explore the energy scalability of the system, the seed input and pulse duration from MA1 were increased to 10 J and 15 ns, respectively, at 1 Hz. The MA2 temperature was decreased to 125 K and the diode pump pulse duration was increased to 1 ms. Output energetics for the experiments are shown in Figure
The dependence of laser performance on the MA2 temperature, diode pump input energy and MA1 seed energy was studied further with the results tabulated in Table
Temp. (K) | Seed (J) | Pump (J) | Energy output (J) | Efficiency (%) |
---|---|---|---|---|
5.6 | 390 | 103.4 | 26.5 | |
5.6 | 390 | 109.7 | 28.1 | |
5.6 | 390 | 112.8 | 28.9 | |
390 | 116.0 | 29.7 | ||
125 | 390 | 120.7 | 30.9 | |
125 | 390 | 124.8 | 32.0 | |
130 | 9.1 | 150.9 | 25.1 | |
125 | 9.1 | 152.4 | 25.4 | |
125 | 10.2 | 153.7 | 25.6 |
Table 1. D100X laser system performance at different temperatures, seed and pump inputs.
3 Conclusion
In summary, we have demonstrated 150 J, 1 Hz nanosecond pulsed output from a cryogenic gas cooled, multi-slab Yb:YAG laser. The system has now exceeded its design specification and is currently being installed in the laser laboratory at XFEL by a joint team of XFEL and CLF engineers and scientists.
References
[1] P. Peyre, R. Fabbro. Opt. Quant. Electron., 27, 1213(1995).
[2] R. P. Drake. Nucl. Fusion, 59(2019).
[3] M. D. Perry, J. A. Sefcik, T. Cowan, S. Hatchett, A. Hunt, M. Moran, D. Pennington, R. Snavely, S. C. Wilks. Rev. Sci. Inst., 70, 265(1999).
[4] T. Tajima, J. M. Dawson. Phys. Rev. Lett., 43, 267(1979).
[5] S. Banerjee, K. Ertel, P. D. Mason, P. J. Phillips, M. De Vido, J. M. Smith, T. J. Butcher, C. Hernandez-Gomez, R. J. S. Greenhalgh, J. L. Collier. Opt. Express, 23, 19542(2015).
[6] P. Mason, M. Divoký, K. Ertel, J. Pilař, T. Butcher, M. Hanuš, S. Banerjee, J. Phillips, J. Smith, M. De Vido, A. Lucianetti, C. Hernandez-Gomez, C. Edwards, T. Mocek, J. Collier. Optica, 4, 438(2017).
[7] O. Novák, T. Miura, M. Smrž, M. Chyla, S. S. Nagisetty, J. Mužík, J. Linnemann, H. Turčičová, V. Jambunathan, O. Slezák, M. SawickaChyla, J. Pilař, S. Bonora, M. Divoký, J. Měsíček, A. Pranovich, P. Sikocinski, J. Huynh, P. Severová, P. Navrátil, D. Vojna, L. Horáčková, K. Mann, A. Lucianetti, A. Endo, D. Rostohar, T. Mocek. Appl. Sci., 5, 637(2015).
[8] P. Vagovič, T. Sato, L. Mikeš, G. Mills, R. Graceffa, F. Mattsson, P. Villanueva-Perez, A. Ershov, T. Faragó, J. Uličný, H. Kirkwood, R. Letrun, R. Mokso, M. Zdora, M. Olbinado, A. Rack, T. Baumbach, J. Schulz, A. Meents, H. Chapman, A. Mancuso. Optica, 6, 1106(2019).
[9] P. D. Mason, S. Banerjee, K. Ertel, P. J. Phillips, T. J. Butcher, J. M. Smith, M. De Vido, S. Tomlinson, O. Chekhlov, W. Shaikh, S. Blake, P. Holligan, M. Divoky, J. Pilar, C. Hernandez-Gomez, R. J. S. Greenhalgh, J. L. Collier. Proc. SPIE, 9513(2015).
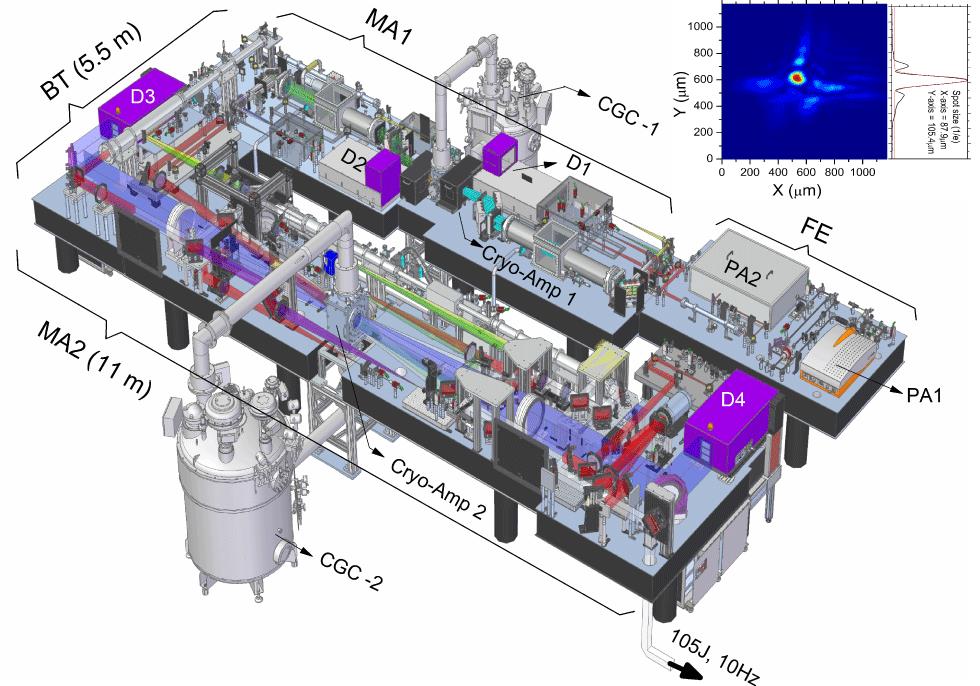
Set citation alerts for the article
Please enter your email address