
- Chinese Optics Letters
- Vol. 21, Issue 4, 043802 (2023)
Abstract
1. Introduction
With the development of chirped-pulse amplification (CPA) technology, numerous petawatt (PW)-class laser facilities have been constructed around the world[1], such as the BELLA laser[2], the J-KAREN-P laser[3], the ELI beamlines[4], and the Shanghai Superintense Ultrafast Laser Facility (SULF) beamlines[5,6]. The PW-class laser can produce focused laser intensities up to
Many techniques have been proposed and developed to improve the temporal contrast of high-power lasers, such as cross-polarized wave generation (XPW)[14], optical parametric amplification (OPA)[15], and plasma mirrors (PMs)[16–20]. Several approaches have been demonstrated in the SULF-1PW beamline before, such as the XPW and OPA filter[6]. Recently, a PM system was developed in the SULF-1PW beamline and tested for a proton acceleration experiment. For the PM, the substrate is usually a polished glass plate that is coated with a broadband antireflection coating[19]. When laser is focused on the surface of the PM, the low intensity pedestal or prepulses pass through the PM substrate with low reflectivity. Then the leading edge of the main pulse would ionize the surface of the PM substrate and generate the high-density plasma[21,22]. Therefore, the subsequent main pulse would be reflected by the high-density plasma surface[23]. The PM provides an ultrafast, self-induced optical switch for temporal contrast enhancement with the unlimited energy and power of the input laser.
In this paper, the characterization of the laser contrast using the PM system in the SULF-1PW beamline is reported. This PM system improves the temporal contrasts on ps and ns time scales by about 2 orders of magnitude. To evaluate the performance of this system, we preliminarily performed proton acceleration contrast experiments. It shows that the proton beams accelerated by the high-contrast (HC, with PM system) laser have significantly improved cutoff energy and quantity compared to the low-contrast (LC, without PM system) case. In addition, simulation studies show that the target with a larger initial expansion scale (due to prepulse) had a lower cutoff energy of the proton beam. These results demonstrate that our PM system effectively improves the laser performance and can be well applied for laser-driven proton accelerations.
Sign up for Chinese Optics Letters TOC. Get the latest issue of Chinese Optics Letters delivered right to you!Sign up now
2. Experimental Setup
The experiments were carried out on the SULF-1PW beamline platform. The schematic of the PM system and the proton acceleration experiment is shown in Fig. 1. In experiments, the laser pulse duration was 27 fs at full width at half-maximum (FWHM), with an 800 nm center wavelength. The p-polarized laser beam was focused by using an f/4 off-axis parabola (OAP1) high-reflection mirror that had a 980 mm focal length with an off-axis angle of 20 deg. The PM substrate was clamped on a three-dimensional motorized translation stage placed in front of the focus position. It should be noted that the PM substrate used in our case was essentially a piece of fused silica glass whose surface was polished and coated with a broadband antireflection coating. The laser transmittance was greater than 99.5% when the PM was not excited. In order to avoid the effect of laser pulse reflected from the rear surface of the PM, the rear surface was also coated with the antireflection coating, and the wedge angle (3 deg) had been designed between the rear and front surfaces of the PM. In addition, the upper and lower parts of the PM were coated with reflective silver to facilitate alignment. After the PM, the divergent beam was recollimated into the parallel beam by OAP2, which had the same parameters as OAP1 and was placed symmetrically with OAP1. Subsequently, the temporal contrast-enhanced laser was reflected by mirror (R3) into the target chamber.
Figure 1.Schematic of the experimental setup. R1–R7 are high-reflection dielectric mirrors; OAP1–OAP3 are off-axis parabola high-reflection mirrors.
The proton acceleration experiments were carried out in the target chamber. As shown in Fig. 1, the laser beam was finally focused by OAP3, which had a 998 mm focal length with an off-axis angle of 26 deg. The 50 nm thickness hydrocarbon target used in the experiment was placed at the laser focus, and the laser incident angle was 30 deg. The radiochromatic film (RCF) stack was placed behind the target at a distance of 80 mm from the target to measure the spatial intensity distribution of the proton beams. It was wrapped with a layer of 14 µm aluminum film to block the X-ray signal. A Thomson spectrometer was placed 420 mm away from the target in the normal direction. It should be noted that the R1 would be removed and R3 would reflect laser directly to target chamber by moving and rotating for the case without the PM.
3. Results
Before measuring the temporal contrast of the laser, it is necessary to find the optimal reflectivity efficiency of the PM used in our case, i.e., to measure the numerical relationship between the intensity of the focal spot on the surface of the PM and the reflectivity. The intensity of laser beam on the PM was varied from
Figure 2.Energy ratio of the reflected and incident laser beams of the PM system.
The temporal contrasts on the ps time scale were measured by employing a high-dynamic-range third-order cross correlator (Sequoia, Amplitude Technologies), while the temporal contrasts on the ns time scale were measured by employing a fast photodiode (ET2030, Electro-Optics Technology) with neutral density filters. The measurement limits for the photodiode and Sequoia are
Figure 3.Measured temporal contrasts without and with PM on the (a) ns time scale and (b) ps time scale.
The comparative studies were made with and without the PM system. The laser energy was fixed to
Figure 4.(a)–(f) Proton beam spatial-intensity distribution for HC (a)–(c) and for LC (d)–(f) collected by the RCF stacks. The small hole in the middle of the RCF lets part of the particles pass through to be measured by ion spectroscopy. (g), (h) Ion imaging trajectories collected by the energy spectrometer for (g) HC and (h) LC laser conditions, respectively; (i) proton energy spectra solved from (g) and (h).
4. Simulation and Discussion
The prepulses can affect the interaction when the main pulse irradiates on targets, because prepulses arrive at the target prior to the main pulse and expand the target. A combination of hydrodynamic codes and particle-in-cell (PIC) simulation to handle the whole interaction driven by prepulses and main pulses has been researched in previous studies[27]. However, for ultrathin solid targets with a thickness of tens of nanometers close to the molecular scale, it is not suitable for hydrodynamic simulations (at the ns scale) anymore[28,29]. Then, a simple model was used to estimate the effect of prepulses in our cases. The interaction of the femtosecond laser with different expanded targets was simulated by using the two-dimensional PIC code (EPOCH[30]).
The laser parameters in the PIC simulations were the same as those used in the experiments. The size of the simulation box is
The energy spectrum from the PIC simulations is shown in Fig. 5. When the initial expanded size of the target was small, the proton cutoff energy was around 14 MeV for the cases of
Figure 5.Energy spectrum of the proton with the different pre-ionized expanded targets from PIC simulations at ∼107 fs after the laser arrived at the front surface of the target. The thickness of the ideal unexpanded target was 50 nm (blue line) with uniform density distribution. The density of the Gaussian function distribution was considered for expanded target cases with thickness (FWHM) of 0.1 µm (green line), 0.5 µm (light blue line), 1.5 µm (red line) and 5 µm (black line).
5. Summary
In conclusion, this paper reports the characterization of the laser contrast using the PM system in the SULF-1PW beamline. This PM system enhances the temporal contrasts on ps and ns time scales by about 2 orders of magnitude. The experiments with proton acceleration also verified that the use of this system can significantly improve the cutoff energy and quantity of proton beams. The simulation can qualitatively predict the expansion of the target with HC and LC lasers. The LC prepulse would greatly increase the expansion of the target, which is bad for proton accelerations. To solve this issue, the PM system is designed to effectively improve the laser contrast in our case. With the progress of PM technology, experiments have shown that PM reflectivity can reach 95%[33], effectively improving the laser energy on target. It is also expected to effectively alleviate the problem of high energy loss in the cascade use of multiple PMs, providing a robust and efficient tool for research on particle acceleration, solid high-order harmonics, and attosecond radiation.
References
[1] C. Danson, D. Hillier, N. Hopps, D. Neely. Petawatt class lasers worldwide. High Power Laser Sci. Eng., 3, e3(2015).
[2] K. Nakamura, H.-S. Mao, A. J. Gonsalves, H. Vincenti, D. E. Mittelberger, J. Daniels, A. Magana, C. Toth, W. P. Leemans. Diagnostics, control and performance parameters for the BELLA high repetition rate petawatt class laser. IEEE J. Quantum Electron., 53, 1200121(2017).
[3] H. Kiriyama, A. S. Pirozhkov, M. Nishiuchi, Y. Fukuda, K. Ogura, A. Sagisaka, Y. Miyasaka, M. Mori, H. Sakaki, N. P. Dover, K. Kondo, J. K. Koga, T. Z. Esirkepov, M. Kando, K. Kondo. High-contrast high-intensity repetitive petawatt laser. Opt. Lett., 43, 2595(2018).
[4] F. Lureau, G. Matras, O. Chalus, C. Derycke, T. Morbieu, C. Radier, O. Casagrande, S. Laux, S. Ricaud, G. Rey, A. Pellegrina, C. Richard, L. Boudjemaa, C. Simon-Boisson, A. Baleanu, R. Banici, A. Gradinariu, C. Caldararu, B. De Boisdeffre, P. Ghenuche, A. Naziru, G. Kolliopoulos, L. Neagu, R. Dabu, I. Dancus, D. Ursescu. High-energy hybrid femtosecond laser system demonstrating 2 × 10 PW capability. High Power Laser Sci. Eng., 8, e43(2020).
[5] W. Li, Z. Gan, L. Yu, C. Wang, Y. Liu, Z. Guo, L. Xu, M. Xu, Y. Hang, Y. Xu, J. Wang, P. Huang, H. Cao, B. Yao, X. Zhang, L. Chen, Y. Tang, S. Li, X. Liu, S. Li, M. He, D. Yin, X. Liang, Y. Leng, R. Li, Z. Xu. 339 J high-energy Ti:sapphire chirped-pulse amplifier for 10 PW laser facility. Opt. Lett., 43, 5681(2018).
[6] Z. Zhang, F. Wu, J. Hu, X. Yang, J. Gui, P. Ji, X. Liu, C. Wang, Y. Liu, X. Lu, Y. Xu, Y. Leng, R. Li, Z. Xu. The 1 PW/0.1 Hz laser beamline in SULF facility. High Power Laser Sci. Eng., 8, e4(2020).
[7] T. J. Yu, S. K. Lee, J. H. Sung, J. W. Yoon, T. M. Jeong, J. Lee. Generation of high-contrast, 30 fs, 1.5 PW laser pulses from chirped-pulse amplification Ti:sapphire laser. Opt. Express, 20, 10807(2012).
[8] J. W. Yoon, Y. G. Kim, I. W. Choi, J. H. Sung, H. W. Lee, S. K. Lee, C. H. Nam. Realization of laser intensity over 1023 W/cm2. Optica, 8, 630(2021).
[9] D.-Q. Wen, P. Zhang, J. Krek, Y. Fu, J. P. Verboncoeur. Higher harmonics in multipactor induced plasma ionization breakdown near a dielectric surface. Phys. Rev. Lett., 129, 045001(2022).
[10] H. Kiriyama, Y. Miyasaka, A. Sagisaka, K. Ogura, M. Nishiuchi, A. S. Pirozhkov, Y. Fukuda, M. Kando, K. Kondo. Experimental investigation on the temporal contrast of pre-pulses by post-pulses in a petawatt laser facility. Opt. Lett., 45, 1100(2020).
[11] L. Ranc, C. Le Blanc, N. Lebas, L. Martin, J. P. Zou, F. Mathieu, C. Radier, S. Ricaud, F. Druon, D. Papadopoulos. Improvement in the temporal contrast in the tens of ps range of the multi-PW Apollon laser front-end. Opt. Lett., 45, 4599(2020).
[12] K. Osvay, M. Csatári, I. N. Ross, A. Persson, C.-G. Wahlström. On the temporal contrast of high intensity femtosecond laser pulses. Laser Part. Beams, 23, 327(2005).
[13] M. Kaluza, J. Schreiber, M. I. Santala, G. D. Tsakiris, K. Eidmann, J. Meyer-ter-Vehn, K. J. Witte. Influence of the laser prepulse on proton acceleration in thin-foil experiments. Phys. Rev. Lett., 93, 045003(2004).
[14] A. Jullien, O. Albert, F. Burgy, G. Hamoniaux, J.-P. Rousseau, J.-P. Chambaret, F. Augé-Rochereau, G. Chériaux, J. Etchepare, N. Minkovski, S. M. Saltiel. 10−10 temporal contrast for femtosecond ultraintense lasers by cross-polarized wave generation. Opt. Lett., 30, 920(2005).
[15] C. Dorrer, I. A. Begishev, A. V. Okishev, J. D. Zuegel. High-contrast optical-parametric amplifier as a front end of high-power laser systems. Opt. Lett., 32, 2143(2007).
[16] C. Thaury, F. Quéré, J. P. Geindre, A. Levy, T. Ceccotti, P. Monot, M. Bougeard, F. Réau, P. d’Oliveira, P. Audebert, R. Marjoribanks, P. Martin. Plasma mirrors for ultrahigh-intensity optics. Nat. Phys., 3, 424(2007).
[17] A. Lévy, T. Ceccotti, P. D’Oliveira, F. Réau, M. Perdrix, F. Quéré, P. Monot, M. Bougeard, H. Lagadec, P. Martin. Double plasma mirro for ultrahigh temporal contrast ultraintense laser pulses. Opt. Lett., 32, 310(2007).
[18] Y. Nomura, L. Veisz, K. Schmid, T. Wittmann, J. Wild, F. Krausz. Time-resolved reflectivity measurements on a plasma mirror with few-cycle laser pulses. New J. Phys., 9, 9(2007).
[19] S. Inoue, K. Maeda, S. Tokita, K. Mori, K. Teramoto, M. Hashida, S. Sakabe. Single plasma mirror providing 104 contrast enhancement and 70% reflectivity for intense femtosecond lasers. Appl. Opt., 55, 5647(2016).
[20] L. Obst, J. Metzkes-Ng, S. Bock, G. E. Cochran, T. E. Cowan, T. Oksenhendler, P. L. Poole, I. Prencipe, M. Rehwald, C. Rödel, H. P. Schlenvoigt, U. Schramm, D. W. Schumacher, T. Ziegler, K. Zeil. On-shot characterization of single plasma mirror temporal contrast improvement. Plasma Phys. Control. Fusion, 60, 054007(2018).
[21] B. Dromey, S. Kar, M. Zepf, P. Foster. The plasma mirror—a subpicosecond optical switch for ultrahigh power lasers. Rev. Sci. Instrum., 75, 645(2004).
[22] I. W. Choi, C. Jeon, S. G. Lee, S. Y. Kim, T. Y. Kim, I. J. Kim, H. W. Lee, J. W. Yoon, J. H. Sung, S. K. Lee, C. H. Nam. Highly efficient double plasma mirror producing ultrahigh-contrast multi-petawatt laser pulses. Opt. Lett., 45, 6342(2020).
[23] X. G. Ge, Y. Fang, S. Yang, W. Wei, F. Liu, P. Yuan, J. Ma, L. Zhao, X. Yuan, J. Zhang. Characterization and application of plasma mirror for ultra-intense femtosecond lasers. Chin. Opt. Lett., 16, 013201(2018).
[24] C. Rödel, M. Heyer, M. Behmke, M. Kübel, O. Jäckel, W. Ziegler, D. Ehrt, M. C. Kaluza, G. G. Paulus. High repetition rate plasma mirror for temporal contrast enhancement of terawatt femtosecond laser pulses by three orders of magnitude. Appl. Phys. B, 103, 295(2011).
[25] G. G. Scott, V. Bagnoud, C. Brabetz, R. J. Clarke, J. S. Green, R. I. Heathcote, H. W. Powell, B. Zielbauer, T. D. Arber, P. McKenna, D. Neely. Optimization of plasma mirror reflectivity and optical quality using double laser pulses. New J. Phys., 17, 033027(2015).
[26] M. H. Xu, Y. T. Li, X. H. Yuan, Q. Z. Yu, S. J. Wang, W. Zhao, X. L. Wen, G. C. Wang, C. Y. Jiao, Y. L. He, S. G. Zhang, X. X. Wang, W. Z. Huang, Y. Q. Gu, J. Zhang. Effects of shock waves on spatial distribution of proton beams in ultrashort laser-foil interactions. Phys. Plasmas, 13, 104507(2006).
[27] W. P. Wang, C. Jiang, H. Dong, X. M. Lu, J. F. Li, R. J. Xu, Y. J. Sun, L. H. Yu, Z. Guo, X. Y. Liang, Y. X. Leng, R. X. Li, Z. Z. Xu. Hollow plasma acceleration driven by a relativistic reflected hollow laser. Phys. Rev. Lett., 125, 034801(2020).
[28] R. Ramis, K. Eidmann, J. Meyer-ter-Vehn, S. Hüller. Multi-fs–a computer code for laser–plasma interaction in the femtosecond regime. Comput. Phys. Commun., 183, 637(2012).
[29] Y. Kuramitsu, T. Minami, T. Hihara, K. Sakai, T. Nishimoto, S. Isayama, Y. T. Liao, K. T. Wu, W. Y. Woon, S. H. Chen, Y. L. Liu, S. M. He, C. Y. Su, M. Ota, S. Egashira, A. Morace, Y. Sakawa, Y. Abe, H. Habara, R. Kodama, L. N. K. Döhl, N. Woolsey, M. Koenig, H. S. Kumar, N. Ohnishi, M. Kanasaki, T. Asai, T. Yamauchi, K. Oda, Ko. Kondo, H. Kiriyama, Y. Fukuda. Robustness of large-area suspended graphene under interaction with intense laser. Sci. Rep., 12, 2346(2022).
[30] T. D. Arber, K. Bennett, C. S. Brady, A. Lawrence-Douglas, M. G. Ramsay, N. J. Sircombe, P. Gillies, R. G. Evans, H. Schmitz, A. R. Bell, C. P. Ridgers. Contemporary particle-in-cell approach to laser-plasma modelling. Plasma Phys. Control. Fusion, 57, 113001(2015).
[31] A. A. Andreev, R. Sonobe, S. Kawata, S. Miyazaki, K. Sakai, K. Miyauchi, T. Kikuchi, K. Platonov, K. Nemoto. Effect of a laser prepulse on fast ion generation in the interaction of ultra-short intense laser pulses with a limited-mass foil target. Plasma Phys. Control. Fusion, 48, 1605(2006).
[32] W. P. Wang, H. Zhang, B. Wu, C. Y. Jiao, Y. C. Wu, B. Zhu, K. G. Dong, W. Hong, Y. Q. Gu, B. F. Shen, Y. Xu, Y. X. Leng, R. X. Li, Z. Z. Xu. Generation of low-divergence megaelectronvolt ion beams from thin foil irradiated with an ultrahigh-contrast laser. Appl. Phys. Lett., 101, 214103(2012).
[33] G. G. Scott, G. F. H. Indorf, M. A. Ennen, P. Forestier-Colleoni, S. J. Hawkes, L. Scaife, M. Sedov, D. R. Symes, C. Thornton, F. Beg, T. Ma, P. McKenna, A. A. Andreev, U. Teubner, D. Neely. Kinematics of femtosecond laser-generated plasma expansion: determination of sub-micron density gradient and collisionality evolution of over-critical laser plasmas. Phys. Plasmas, 28, 093109(2021).
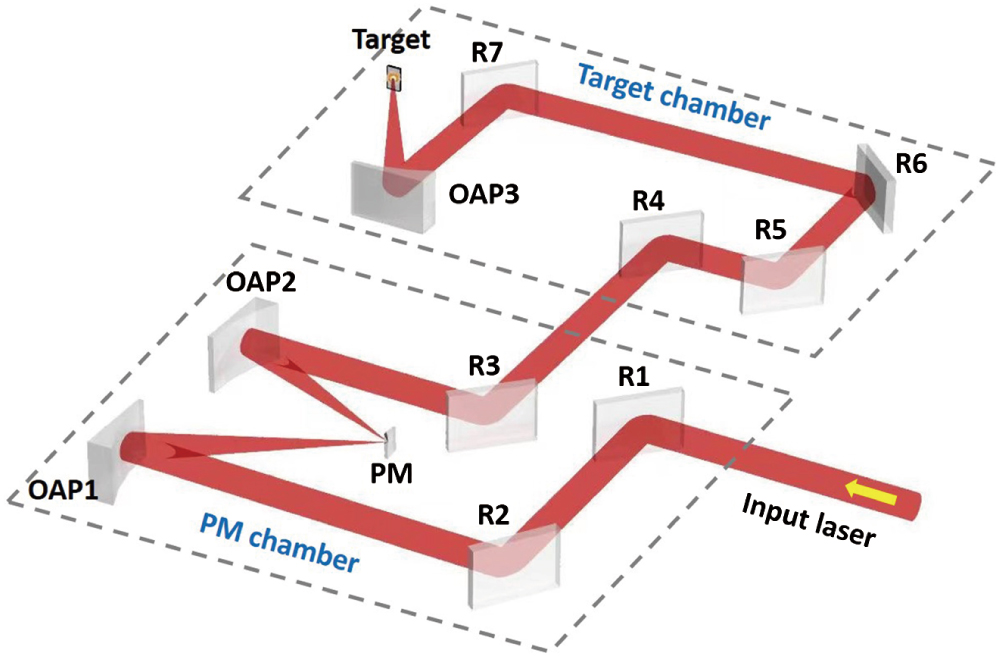
Set citation alerts for the article
Please enter your email address