Monette H. Khadr* and Hany Elgala
Abstract
A plethora of physical-layer techniques aim to enhance the performance of communication systems in several ways. Spectral efficiency and security are on the top of the list of enhancements; however, both are isolated and antagonistic islands of research. Augmented communication (ACom) is introduced in this Letter as the first technique that aims to combine these two enhancements in visible light communications (VLCs). The dividends of the proposed concept are demonstrated via simulations and the performance is experimentally validated. Results show that ACom can simultaneously provide the high spectral efficiency and the resistance to eavesdropping, while introducing minimal signal-to-noise ratio penalties.Sixth generation (6G) communication networks will most likely adopt carrier frequencies that are beyond 30 GHz to exploit the wide available bandwidth in order to accommodate the insatiable demand for high data rates[1]. Visible light communications (VLCs) shift the communication frequency to the visible spectrum by utilizing the pre-existing lighting infrastructure and are emerging as a strong contender for future high-speed networks[2]. Not only does VLC technology promise to alleviate the current congestion problem in the radio frequency (RF) range, the propagation properties of light give it a huge advantage. Since light cannot penetrate opaque surfaces, the communication bandwidth can be reused across multiple rooms, thus it has a high area spectral efficiency, and physical-layer (PHY) security is achieved as the information is confined within one room. However, in practical scenarios, eavesdroppers can be co-located with legitimate users in the same room. Hence, further measures need to be taken.
For spectral efficiency enhancement, higher modulation orders and efficient orthogonal frequency division multiplexing (OFDM) are considered[3]. An alternative approach is based on multiple antenna configurations to enable spatial-domain multiplexing and beamforming[4]. However, the performance of these techniques relies heavily on the rich scattering nature of the channel and the accuracy of channel state information (CSI). Due to the properties of the optical channel, it is extremely challenging to apply multiple-input multiple-output (MIMO) in VLC. The nature of the channel enforces an ill-conditioned (almost singular) channel matrix that prohibits spatial decorrelation. Hence, these schemes are channel dependent and require systems with a high complexity. With the rise of new Internet-of-Things (IoT) applications, systems cannot withstand additional complexity. Hence, the focus has reverted to improving the spectral efficiency of pre-existing OFDM techniques.
On the other hand, PHY security schemes are proposed as an appealing approach to enhance the robustness of communication systems by complimenting cryptographic methods. These schemes include hierarchical modulation, coding, fingerprinting, wireless channel exploitation, and jamming[5]. Most security techniques sacrifice spectral efficiency for the sake of providing security, require additional resources, or are tailored for channels that are spatially distinct, which is not the case for systems operating beyond the RF range, such as in VLC[6].
Sign up for Chinese Optics Letters TOC. Get the latest issue of Chinese Optics Letters delivered right to you!Sign up now
In this Letter, augmented communication (ACom) is introduced as a concept to utilize the PHY in its full potential toward the goal of realizing a high spectral efficiency and secure links by design. Given the importance of these two features, i.e., spectral efficiency and security, ACom presents for the first time (according to the authors’ knowledge) a system that can combine both. ACom aims to design new modulating waveforms by rethinking and preconditioning the in-phase and quadrature (IQ) values of conventional baseband streams. The resulting waveforms attain novel features without relying on the CSI, coding, and knowledge of the eavesdropper’s location nor requiring multiple antenna configurations. In this work, as an initiatory analysis of ACom, the investigation of optical OFDM for VLC is introduced. The focus is on demonstrating the added spectral efficiency improvement and the PHY security feature. Simulation and experiments are conducted to quantify the minimal degradation in the bit-error rate (BER) performance of a legitimate VLC user and the insignificant signal-to-noise ratio (SNR) penalty associated with ACom.
In VLC, a light emitting diode (LED) and a laser diode (LD) are the two main types of optical sources used. Most VLC systems are non-coherent, i.e., the information is conveyed in the intensity of the emitted light. These systems are known as intensity-modulation direct-detection (IM/DD) systems, as the received signal is directly detected using a photodetector, i.e., a photodiode (PD), without the need for a local oscillator. OFDM has been widely adopted in VLC due to its frequency domain equalization simplicity and its ability to achieve Shannon capacity with the use of suitable bit/power loading schemes[7]. Conventional OFDM waveforms used in RF systems cannot be directly applied in IM/DD systems. Since IM/DD systems rely on intensities, the signal must be real and non-negative. The two most eminent optical OFDM techniques are direct current optical OFDM (DCO-OFDM) and asymmetrically clipped optical OFDM (ACO-OFDM). These techniques impose Hermitian symmetry (HS) before performing the inverse fast Fourier transform (IFFT) operation, which results in a loss of spectral efficiency. In DCO-OFDM, only half of the OFDM subcarriers are carrying information, while in ACO-OFDM only a quarter. ACO-OFDM sacrifices the spectral efficiency to preserve the power efficiency.
For the implementation of PHY security to combat eavesdropping attacks from eavesdroppers co-located with legitimate VLC users in the same room, separate solutions were proposed. However, most of them require the knowledge of the eavesdropper’s location, such as in Ref. [8], which maybe unfeasible in practical scenarios. Another solution builds an eavesdropper-free zone around the access points[2]. Using motion sensors, the access point became aware if an eavesdropper entered the eavesdropper-free zone. However, the system responded by temporarily stopping the communication with the legitimate user.
In ACom, the transmitted waveform is preconditioned by design through augmented modulation (AMod), i.e., an augmented modulator. Hence, the receiver excerpts two data streams from the received waveform. The modulation stream is associated with reverting conventional IQ-samples to their default form and recovering IQ-symbols using a conventional demodulator. The second stream is the augmented stream and is conveyed with AMod. The augmented stream is recovered through augmented demodulation (ADeMod), i.e., an augmented demodulator. Only a predesignated receiver of a legitimate user can detect and estimate the alterations made through the preconditioning step (see Fig. 1). Thus, both the spectral efficiency and the security gains can be simultaneously harvested. ACom exceeds the spectral efficiency performance of DCO-OFDM and reserves the confidentiality of the transmitted signal without the need for additional hardware (i.e., sensors) or the knowledge of the eavesdropper location. Conceptually, the proposed ACom concept could be applied on any single- or multi-carrier modulation technique. However, in this work, the system model is built on top of OFDM system as it is the de facto modulation and multiple access scheme used in most current standards.
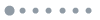
Figure 1.Block diagram detailing an ACom-based VLC system using OFDM, depicting the transmitter, receiver, and the optical channel in between.
An OFDM signal is comprised of the sum of independent quadrature amplitude modulation (QAM) sub-signals with representing the number of active subcarriers. A vector of QAM constellation points is transformed via IFFT into the discrete-time vector , where the discrete-time index denotes the number of Nyquist rate samples and superfix is the transpose operation. In HS IM/DD VLC, for to be real, and must be real and the following constraint must be satisfied[9]where denotes the complex conjugate. Hence, only elements are specified in the QAM vector. The transmitted ACom signal in its general form is where is the function representing the augmented modulator. Figure 1 shows the block diagram of the proposed ACom system, with denoting the additive white Gaussian noise (AWGN) and is the received vector. Since the two dominant sources of noise are the shot and thermal noises, an AWGN channel model is an appropriate presentation of a VLC channel. One possible realization, which is the analysis in this Letter, is the case where is a linear function and can be represented by where and are designed scaling factors based on the dynamic range of the light source and is a binary sequence with Figure 2 illustrates an example of an ACom signal showing the effect of the various parameters on the construction of the transmitted signal . As depicted in Fig. 2, is divided into chunks, with bits in taking identical values and the parameter Values of the scaling factors, and , are limited by the characteristics of the transceiver frontends, i.e., light sources, power amplifiers, and data converters. Hence, additional information can be transmitted by varying the states of giving possible combinations per OFDM symbol. In our initial design, the set of all possible alterations made by the transmitter through preconditioning is assumed to be known at the receiver, and thus the alterations become a deterministic function that can be estimated. In this given system, ACom increases the number of transmitted bits per OFDM symbol to become where represents the utilized QAM modulation order. The maximum attainable number of bits for this realization is . Hence, the spectral efficiency of ACom using the function specified in Eq. (4) and the condition when , , can be calculated as . For illustration, using the equations stated above, assuming a 64-IFFT OFDM-based binary phase-shift keying (BPSK) system with a bandwidth equal to 20 MHz, the throughput of a DCO-OFDM system would be 10 Mbps, while that of an ACom-based DCO-OFDM system is 30 Mbps. Table 1 compares the spectral efficiency of ACom with eminent HS-based optical OFDM techniques presented in literature.
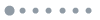
Figure 2.Example of the time-domain representation of an ACom signal, with an IFFT length of 16. The construction is showing the parameters and and their effect on the generated signal for a specific .
OFDM Technique | Spectral Efficiency |
---|
ACO-OFDM | N/4log2M |
DCO-OFDM | N/2log2M |
eU-OFDM[11] | ≤N/2log2M |
SEE-OFDM[12] | 3N/8log2M |
ACom | ≤N/2log2M+N |
Table 1. Spectral Efficiency of Optical OFDM Techniques
According to the central limit theorem, the subchannel elements can be seen as Gaussian independent and identically distributed (i.i.d.) random variables[10], and hence is an i.i.d. Gaussian random process. Since is a linear transformation of , the probability density function (PDF) of will follow that of , and hence is a Gaussian random process. The PDF is where with mean and variance . Hence, the PDF of the transmitted signal is where is the average transmitted energy per QAM symbol and the transmitted power now becomes
The analytical BER expression for -QAM signaling in AWGN can be approximated as[13]where the -function is defined as given is the cumulative distribution function of the normal Gaussian distribution. To quantify the security gains of ACom, a metric associated with the secrecy BER, , is established. It can be defined as the maximum achievable BER by an eavesdropper trying to intercept the transmission between legitimate users. The effective SNR for the transmitted signal is where is the OFDM symbol energy scaled by and . Thus, the term represents the SNR penalty cost. It is important to note that the value of parameter affects the amplitude of the OFDM’s peaks, which in return affects the peak-to-average-power-ratio performance. However, according to the previous analysis in Ref. [14], choosing only results in less than a 1% increase in the peak power. At the receiver’s end, first an ACom receiver performs hypothesis testing in order to estimate . There are hypotheses, denoted by , with corresponding to the possible binary sequences.
Defining as auxiliary vectors given by , where . Conditioned on , and the corresponding estimates , the joint PDF where is the variance of . Given the received signal the receiver will return the hypothesis that maximizes Eq. (13), i.e.,
Once is known, it is passed to the augmented demodulator to extract the augmented data stream, while the conventional OFDM receiver demodulates , which is the received signal after eliminating (see Fig. 1). An eavesdropper, on the other hand, lacks the knowledge of these hypotheses and thus will not be able to revert the signal to its original form.
Figure 3(a) shows the spectral efficiency gain of utilizing ACom, showing that it provides an enticing spectral efficiency gain as a function of the QAM order. The zero value on the y axis of Figs. 3(a) and 3(c) denotes the spectral efficiency of conventional DCO-OFDM as a benchmark. From Fig. 3(a), ACom can triple the spectral efficiency of a BPSK OFDM-based system. In the case of 4-QAM, it is increased by 122%, i.e., more than doubled. This interesting gain can allow transmission at lower modulation orders to suit possible channel degradation while maintaining high data rates. Moreover, the spectral efficiency can be increased by approximately 25% at modulation order as high as 256. Additionally, the spectral efficiency gain of ACom also varies with the IFFT length. Figure 3(b) shows the significant improvement in spectral efficiency especially for higher IFFT lengths at when compared to DCO-OFDM transmission. As shown in Fig. 3(b), the gain is proportional to the IFFT length. Based on these notable findings, ACom is showing its potential as a propitious candidate for meeting future data-rate requirements. Even though the maximum spectral efficiency occurs at , an analysis on applying smaller values of is given in Fig. 3(c). It can be observed that even by utilizing the simplest realizations, for example at , a considerable gain can still be earned, with a 20% increase for BPSK and a 10% increase for 4-QAM.
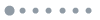
Figure 3.Simulation results depicting the relative spectral efficiency gain of ACom versus various system parameters. (a) Modulation order at , (b) IFFT length at and , and (c) for various .
Figure 4 shows the effect of varying the parameter as a function of the SNR in the presence of AWGN on the BER performance of the OFDM stream for both the legitimate user and the eavesdropper. The simulation uses 64-QAM and an IFFT length of 64. The performance of a legitimate user is almost identical to the analytical model obtained from Eq. (10), showing a minimal BER degradation caused by the induced reduction. For the eavesdropper, the performance is drastically different. As shown in Fig. 4, the saturates at a value dependent on . As increases, starts to saturate faster, reaching at an for . The repercussion of varying on the effective SNR is studied and presented in Fig. 5; as observed, at , the effective SNR penalty is about 0.3 dB. The experimental hardware setup is implemented using a USRP-N210[15] with integrated LFTX and LFRX daughterboards, which is connected to an Intel NUC with an i7-7567U processor, with installed MATLAB 2018b for signal generation and processing, as shown in Fig. 6.
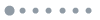
Figure 4.BER versus SNR performance with the solid red line denoting the analytical performance and the remaining curves showing ACom at different for both the legitimate user (User) and the eavesdropper (Eve) at .
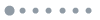
Figure 5.Effective SNR reduction versus showing that at the effective SNR is reduced to only be 0.3 dB.
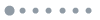
Figure 6.Experimental setup of ACom using a USRP-N210 with two integrated daughterboards showing the optical frontends and the NUC used for ACom signal processing.
Besides LEDs, LDs have been extensively used in experimental VLC system deployments[16], and hence, in our demonstration, an LD is used. Our optical frontend is composed of a Thorlabs HL63163DG LD and a PDA10A positive-intrinsic-negative (PIN) PD. The LD is biased to about 70 mA using a TCLDM9 mount and is attached to current and temperature controllers. Fresnel lenses with a 25 mm focal length are used to control the collimating emission and to focus the received beam of light on the active area of the considered photodetector. The signal originates from the NUC and passes to the LFTX daughterboard, which sends the ACom symbols to the transmitter frontend. The PIN PD at the receiver frontend forwards the received ACom symbols to the LFRX daughterboard and then back to the NUC for processing. The NUC and the USRP are connected via an Ethernet cable. In our implementation, each eight consecutive OFDM symbols were packed to form an OFDM frame. A preamble is added at the beginning of each frame to allow frame synchronization. Figure 7 shows the experimental legitimate user OFDM stream BER performance of ACom using 4-QAM at various values, with a separation distance of 50 cm between the LD and PD. To measure the performance versus various SNR levels, in addition to the experimentally measured noise, AWGN is added to the transmitted signal using MATLAB communication toolbox and is fed to the LD. For simplicity of analysis, and are normalized in MATLAB, and thus according to the relationship . As shown in Fig. 7, the SNR penalty is insignificant and is consistent with the results obtained via simulations. To quantify the impact of parameter on the security dividends of ACom, the eavesdropper’s BER is evaluated experimentally and presented in Fig. 8. The results show that the eavesdropper’s BER degrades with the increase of . The eavesdropper’s BER slightly varies with , yet as shown in Fig. 8, smaller values of increase the eavesdropper’s BER. However, in all cases the eavesdropper’s BER is limited to the range.
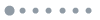
Figure 7.Experimental BER performance at 4-QAM with an IFFT length of 64 for the legitimate user at where is equivalent to conventional DCO-OFDM transmission.
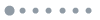
Figure 8.Experimental BER performance of the eavesdropper as a function of and at an SNR level of 20 dB at 4-QAM with an IFFT length of 64.
In conclusion, ACom was introduced as a perception to increase the spectral efficiency while adding PHY security without entailing any additional resources. Simulation results proved the potential of ACom, as it was able to achieve a 25%–200% spectral efficiency gain in comparison to conventional DCO-OFDM-based systems. It can allow transmission at lower modulation orders to suit possible channel degradation while maintaining high data rates. ACom can saturate the detection capability of an eavesdropper to a BER in the range of with an insignificant SNR penalty of around 0.3 dB. Experimental demonstration verified the simulation analysis.
References
[1] A. Shahmansoori, G. E. Garcia, G. Destino, G. Seco-Granados, H. Wymeersch. IEEE Trans. Wireless Commun., 17, 1822(2018).
[2] L. Yin, H. Haas. IEEE J. Sel. Areas Commun., 36, 162(2018).
[3] M. S. Hossain, T. Shimamura. IEEE Commun. Lett., 20, 2249(2016).
[4] S. Gao, M. Zhang, X. Cheng. IEEE Trans. Wireless Commun., 17, 17(2018).
[5] F. Jameel, S. Wyne, G. Kaddoum, T. Q. Duong. IEEE Commun. Surv. Tutorials, 21, 2734(2019).
[6] J. M. Hamamreh, H. M. Furqan, H. Arslan. IEEE Commun. Surv. Tutorials, 21, 1773(2019).
[7] A. Barbieri, G. Colavolpe, T. Foggi, E. Forestieri, G. Prati. J. Lightwave Technol., 28, 2537(2010).
[8] A. Mostafa, L. Lampe. IEEE Trans. Signal Process., 64, 6501(2016).
[9] J. Tellado, L. M. C. Hoo, J. M. Cioffi. IEEE Trans. Commun., 51, 218(2003).
[10] L. Yang, X. Lin, X. Ma, K. Song. IEEE Commun. Lett., 22, 2156(2018).
[11] D. Tsonev, H. Haas(2014).
[12] H. Elgala, T. D. C. Little(2014).
[13] Y. S. Cho, J. Kim, W. Y. Yang, C. G. Kang. MIMO-OFDM Wireless Communications with MATLAB(2010).
[14] M. H. Khadr, H. Elgala, M. Ayyash, T. Little, M. Rahaim, A. Khreishah(2019).
[15]
[16] I. Lu, C. Yeh, D. Hsu, C. Chow. IEEE Photon. J., 8, 7904106(2016).