X. Zhu1,†, S. Chen2,†, M. Zhang1,*, L. Chen2,3,5..., Q. Wu1, J. Zhao4, Q. Jiang1, Z. Zheng1 and H. Zhang2|Show fewer author(s)
Abstract
We fabricate titanium disulfide () using a liquid exfoliation method and subsequently a -based device by optically depositing the material onto the microfiber. This device exhibits a strong nonlinear saturable absorption property with an optical modulation depth of 8.3% at 1560 nm. With the implementation of this all-fiber -based saturable absorber, we demonstrate that both mode-locking and -switching operation can be obtained in a turn-key all-fiber erbium-doped laser cavity. Our findings constitute the first example, to the best of our knowledge, of a -based saturable absorber for ultrashort pulse generation and highlight the great potential of such devices in two-dimensional nanomaterials-related photonics.1. INTRODUCTION
The ultrafast fiber laser is a powerful technology used in a wide range of scientific, industrial, and biomedical applications, e.g., micromachining, telecommunication and medical imaging. While there are various approaches to generate ultrashort pulses in fiber lasers, a commonly used method is mode-locking (or -switching) with a saturable absorber (SA), which acts as a passive optical switch to modulate intracavity loss and enables access to a wide space of pulse parameters. An early study has shown the demonstration of a dye SA to obtain the mode-locking performance in a Nd:fiber laser but with low stability [1,2]. In the 1990s, semiconductor saturable absorber mirrors (SESAMs) were proposed to achieve stable mode-locking and have subsequently developed into a mature technology for ultrafast pulse generation [3]. Although SESAMs have been successfully and widely used in various operational ranges of mode-locked lasers, they still suffer from limitations, including narrow operating bandwidth, limited relaxation speed (normally ), and high fabrication expense. To address these challenges, there is proliferating research in the exploration of alternative materials for SA applications.
Recently, low-dimensional nanomaterials have attracted intense interest due to their remarkable optical and optoelectronic properties [4–11]. One-dimensional (1D) carbon nanotubes have been demonstrated as a promising SA candidate for pulse generation, exhibiting nonlinear optical properties and subpicosecond relaxation times. The flexible implementation of this material offers new construction approaches for fiber lasers, e.g., direct coating on the fiber end or using the light–matter interaction between the material and fiber mode via evanescent field coupling. Two-dimensional (2D) materials, such as mono- or few-layer graphene, topological insulators, transition metal dichalcogenides (TMDs) [1,6–9], and black phosphorus [10–13], have also emerged with excellent features, such as high third-order optical nonlinear susceptibility and ultrafast carrier dynamics, providing exciting opportunities for optical applications.
TMDs are a family of more than 40 different layered nanomaterials and have a general chemical structure of , in which is a transition metal atom (e.g., Mo, ) and a chalcogen atom (e.g., , Se). They may behave as metallic, semiconducting, or insulating, depending on the coordination and oxidation states of the atoms [14]. TMDs have been exfoliated into single- and few-layer forms because of the relatively weak van der Waals force that binds individual layers in TMD bulk crystals, leading to different optoelectronic properties since these properties are highly thickness dependent [1,8]. For instance, the bandgap of TMDs shifts from bulk material (indirect) to monolayer form (direct), typically ranging from to 2 eV. Such layer-dependent behavior, along with other characteristics including robustness and environmental stability, make TMDs attractive for a variety of photonics and optoelectronics applications. In particular, the remarkable optical properties of TMDs have led to the demonstration of numerous nonlinear optical phenomena. A number of studies exploiting the saturable absorption property of several sulfide- and selenide-based TMDs (such as , , , using different deposition techniques, including microfiber-based devices and embedding the few-layer nanomaterial in transparent polymer films) have been reported to produce ultrashort pulses in laser systems. The operating wavelength region (e.g., either at discrete wavelengths or with broadband tunability) of the reported TMDs in the literature is below their fundamental bandgaps, which can be attributed to the subbandgap saturable absorption of these materials.
More recently, titanium disulfide (), another prototypical layered nanomaterial, has emerged as a candidate material for potential photonic and optoelectronic applications. It has been successfully demonstrated in a variety of applications, including photovoltaic systems and photoelectrochemical hydrogen generation [15]. While the literature has shown that many conflicting results exist on the electronic structure of bulk , the bandgap structure of this material has been experimentally reported to exhibit semiconducting properties, covering a wide range of the spectral region (0.05–2.5 eV) [16]. Although could offer advantages over many 2D materials for certain applications, e.g., where a broad energy bandgap is required, its optical properties, in particular, nonlinear optical response, have yet to be fully studied. Therefore, it is desirable to further investigate the nonlinear optical properties of and to exploit it in practical systems for potential SA applications.
Here, we report a microfiber-based few-layer -based SA for ultrashort pulse generation. It is fabricated by using an optical deposition method and exhibits strong nonlinear response at 1.56 μm. Using this -based SA device in an erbium-doped (Er-doped) fiber laser, we demonstrate stable mode-locked and -switched operation at 1.56 μm. This has enabled us to underscore the applicability of as a new viable SA candidate and to explore more photonics applications.
2. RESULTS AND DISCUSSION
A. Fabrication and Characterization of the TiS2 Material
Liquid-phase exfoliation refers to breaking a layered crystal into 2D materials by suspending the crystal in a “solvent” and blasting the crystal with ultrasonic energy [17]. Liquid-phase exfoliation is a highly effective approach for preparing large-scale and highly concentrated atomically thin 2D material nanosheets [18]. The general process for preparing ultrathin and lager scale flakes by liquid-phase exfoliation involves three steps: (i) dispersion of the starting material in a stripping solvent (to exfoliate the ), (ii) exfoliation via sonication, and (iii) centrifugation. The few-layer is exfoliated from the bulk crystals using liquid-phase exfoliation in -methyl-2-pyrrolidone (NMP) [10] solvent, an approach commonly used to produce large-scale exfoliation of materials and uniform dispersion in the exfoliation medium [18]. Briefly, 20 mg of the bulk powder (purchased from Aladdin) is mixed with 20 mL saturated NaOH NMP solution by sonicating at 40 kHz frequency and 400 W power for 5 h. During the ultrasonic process, was treated with an ice bath to keep its temperature below 25°C. After sonication, the in NMP is separated and transferred to water by centrifugation (14,000 r/min, 30 min).
In order to confirm that the was successfully exfoliated into ultrathin nanosheets, we characterized the nanosheets by employing transmission electron microscopy (TEM) and atomic force microscopy (AFM). The appropriate concentration of the ethanol solution droplets on the copper was meshed for preparation of the TEM sample. As shown in Fig. 1(a), the prepared few-layer can be clearly identified with a layered structure of several hundreds of nanometers, indicating that we have successfully prepared the few-layer nanosheets by liquid-phase exfoliation. Samples for AFM are prepared by drop-casting diluted dispersions onto a wafer and drying to remove the organic solvent. As shown in Fig. 1(c), the morphology of is typical flakes and the size of the nanosheets is about 200 nm. The height profile of the nanosheet, as shown in Fig. 1(d), suggests that the thickness of nanosheets marked in Fig. 1(c) is 2 nm. It proves that we have successfully prepared ultrathin few-layer nanosheets with a thickness of 2 nm, corresponding to layers. We then measured the Raman spectra of nanosheets by using the Horiba Jobin–Yvon LabRam HR-VIS high-resolution confocal Raman microscope equipped with a 633 nm laser as the excitation source. As shown in Fig. 1(b), the identity of the can be verified by four characteristic peaks located at 176.5, 220.3, 333.3, and corresponding to , , , and vibration modes of , respectively. This is consistent with values reported in Refs. [16,19,20].
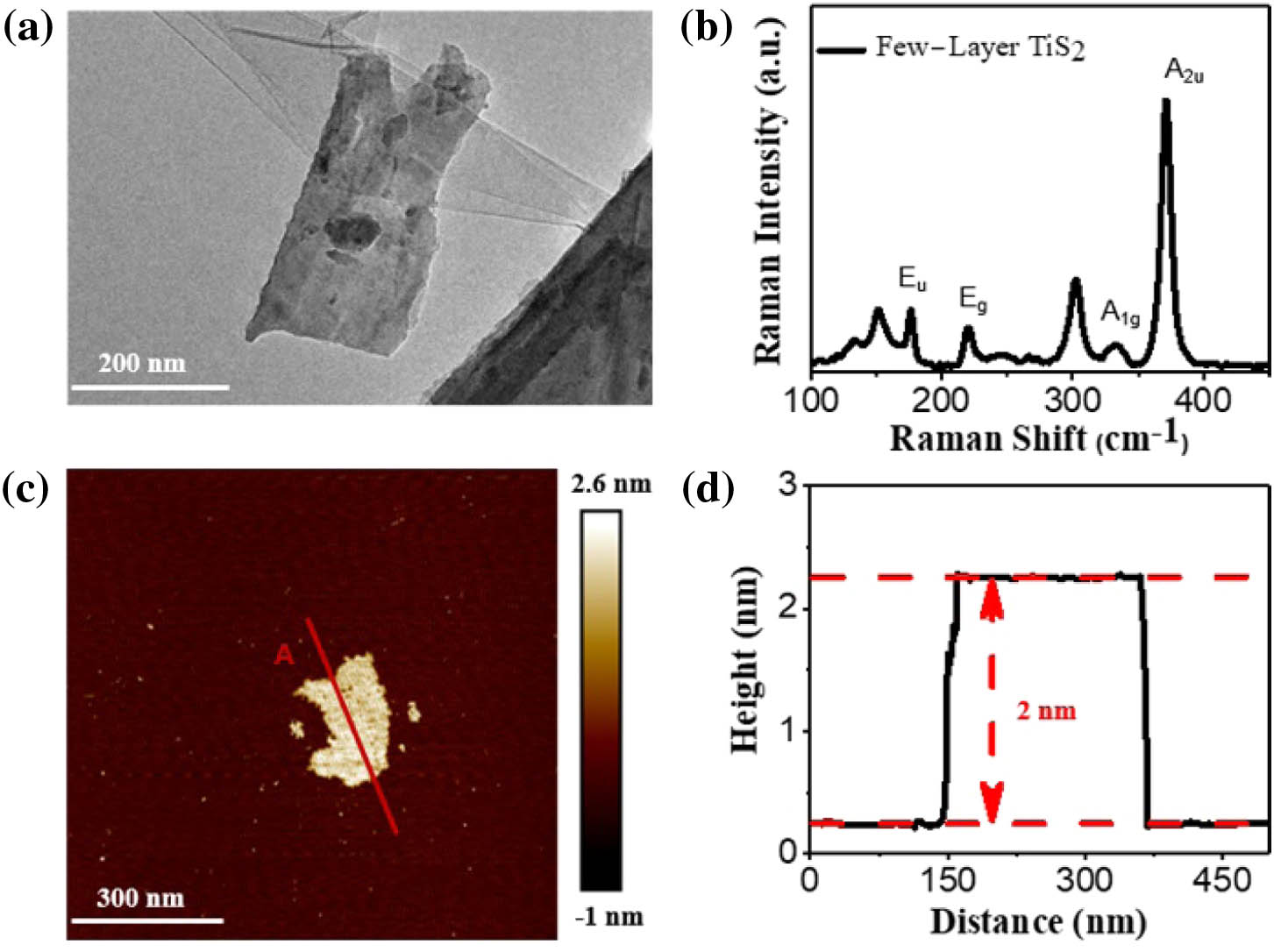
Figure 1.Characterization of the few-layer nanosheets: (a) TEM image of the nanosheets; (b) corresponding Raman spectrum of few-layer nanosheets; (c) AFM image of few-layer nanosheets; (d) height profile of the section marked in (c).
In our experiment, the -based SAs used for short pulse generation are realized by the nonlinear interaction of the processed material with the evanescent field of light in a microfiber. The microfiber is fabricated by polishing a single-mode fiber after holding with an arcuate block. An optical power meter is used to monitor the insertion loss, which indicates the space between the fiber core and the polished surface. The waist diameter and the polished length are measured to be and , respectively. The insertion loss of the integrated microfiber, measured with a continuous wave laser centered at 1.55 μm, is 0.4 dB. The microfiber-based -based SA is fabricated using the optical deposition method, an approach similar to other layered nanomaterial-based SA devices via evanescent field mediating the strength of the light–matter interaction on tapered or -shaped fibers. The solution is dropped on the cross section of the microfiber fixed on a quartz plate. A 980 nm continuous-wave laser with optical power of 60 mW is then coupled into the microfiber and initiates the optical deposition process. The light propagating through the microfiber device is collected by an optical power meter to evaluate the deposition depth in real time [Fig. 2(a)].
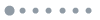
Figure 2.(a) Diagram of fabricating the microfiber-based using an optical deposition method; (b) saturable absorption property of the SA device.
The nonlinear optical absorption of the -based SA is characterized using a power-dependent transmission technique based on a balanced twin-detector measurement [21]. A home-built fiber laser system (with 500 fs pulse duration, 1.56 μm wavelength, 20 MHz repetition rate) is used as the pump source, where the output is split using a 50:50 fused fiber coupler, the latter enabling monitoring of power as a reference signal for normalization. By continuously adjusting the attenuator, the transmitted power is recorded as a function of the incident optical power on the microfiber-based -based SA device. From the measurement, we found our sample exhibited a strong saturable absorption response that is well-fitted by the two-level SA model [22]. The representative plot is shown in Fig. 2(b), with the parameters extracted from the fit results: the saturation power, , and modulation depth (maximum value of the transmission shifted to be 100% but without normalization), 8.3%, all of which are similar in magnitude to previously reported saturable absorbers based on other 2D nanomaterials [6,8,14]. The strong saturable absorption property of the microfiber-based -based SA at 1.56 μm indicates that this device could be used as an ultrafast optical switch for ultrashort pulse generation in an Er-doped fiber laser.
B. Demonstration of Ultrashort Pulse Generation in a Fiber Laser Using a TiS2-Based SA
An Er-doped fiber laser is constructed using all single-mode fiber-integrated components for an alignment-free, compact, and low-cost system, as shown in Fig. 3(a). A ring cavity design is adopted, including a polarization-independent optical isolator to ensure unidirectional propagation, a 50:50 fused fiber output coupler for both temporal and spectral diagnostics, and a polarization controller enabling a thorough and continuous adjustment of the net cavity birefringence, in addition to the fiber amplifier. The fiber amplifier consists of a length of 0.7 m Er-doped active fiber (LIEKKI Er80-8/125, with a group velocity dispersion of ), copumped by a 980 nm laser diode. The total cavity length is and the net cavity dispersion is calculated to be , ensuring laser operation in the average-soliton regime [23].
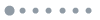
Figure 3.(a) Configuration of the Er-doped fiber laser for mode-locking operation; (b) output pulse train; (c) optical spectrum; (d) radio frequency spectra, where and the higher harmonics on a span of 500 MHz; (e) the intensity autocorrelation profile of the generated pulses.
Self-starting mode-locking is observed from the fiber laser cavity, generating a steady train of pulses at the fundamental repetition frequency of the laser cavity of 22.7 MHz [Fig. 3(b)] with a single pulse energy of 25.3 pJ. The spectrum of the output pulses is centered at 1563.3 nm, with a full width at half-maximum (FWHM) of 4.75 nm [Fig. 3(c)]. The radio frequency (RF) spectra, which could be used to infer the laser stability, are shown in Fig. 3(d). The fundamental RF shows a signal-to-background extinction ratio, indicating relatively low-amplitude fluctuations. The inset of Fig. 3(d) shows higher cavity harmonics without any noticeable sign of -switching instabilities (on a span of 500 MHz), implying stable mode-locking operation performance. The temporal profile of the output pulses is characterized using an intensity autocorrelator. As shown in Fig. 3(e), the plot is well-fitted with a pulse shape, with a duration of 812 fs (deconvolved). The time–bandwidth product is calculated to be .
As the typical characteristics of soliton lasers, soliton sidebands arising from resonances between the soliton and the dispersive wave components emitted after soliton perturbations can be observed in the output of the spectrum. In our experiment, despite the laser consisting of nonpolarization discrimination components, the position of one set of the spectral sidebands shifts when deliberately adjusting the PC while the other set remains unchanged. This indicates the formation of a vector soliton in the fiber laser. To identify the differences of the narrow peaks superimposed on the soliton spectrum, the output light is separated into two orthogonal polarization components using an external PC, which balances the fiber-pigtail-induced linear polarization rotation, followed by a fiber pigtailed external cavity polarization beam splitter.
Figure 4(a) shows the polarization-resolved spectra of the solitons, with a green line and a red line representing the vertical axis and the horizontal axis, respectively. It is noted that one set of the spectral sidebands displays either as a peak or a dip (labeled with arrows) between two orthogonal polarization components while the soliton spectral sidebands always exhibit as peaks. The appearance of such a peak-dip relationship indicates the existence of coherent energy change between two soliton components [24]. The oscilloscope traces of the polarization-resolved pulses are shown in Fig. 4(b). All pulses in the cavity have the same amplitude, implying that polarization-locked vector solitons were formed. This can be explained by the fact that pulses are under intracavity birefringence with a locked group velocity and phase velocity. The nonlinear birefringence induced by the nonlinear effects, e.g., self-phase modulation, cross-phase modulation and four-wave mixing coherent energy coupling, compensates the intrinsic birefringence, and as such solitons propagating in the laser cavity could maintain their own polarization states [25].
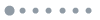
Figure 4.(a) Polarization-resolved spectra of the solitons, with a green line and a red line representing the vertical axis and the horizontal axis, respectively; (b) the total and polarization-resolved soliton pulse trains.
In addition to mode-locking, the inclusion of an SA in a fiber laser cavity can also initiate -switching, an important technique modulating the cavity factor to periodically emit light as a pulse train with a kilohertz repetition rate and nanosecond to microsecond durations. Typically, the implementation of an SA into a fiber laser can initiate both mode-locking and -switching. The transition between the two pulsating regimes could be mediated by a balance between gain and loss, which can be controlled by the optimization of the pump power, the careful design of the nonlinear optical properties of the ultrafast optical switch (SA devices), and the management of the cavity dispersion map. In our cavity, -switched emission can be achieved by a thorough adjustment of the intracavity birefringence using the polarization controller, where the wavelength position of the spectral loss induced by the birefringence filter can be tuned in addition to the optimization of the intracavity optical power. Typical pulse characteristics of the -switched operation are summarized in Fig. 5. The represented -switched pulses, centered at 1560.2 nm, are generated at 33.387 kHz repetition rate, with an FWHM pulse duration of 4.01 μs [Figs. 5(a) and 5(b)]. The RF spectrum of the output shows a signal-to-background contrast of 40.56 dB, which is comparable to the reported -switched operation achieved using other TMD-based SAs in the literature [1,8,26–29]. The pulse properties in continuous-wave (CW) pumped -switched lasers rely on the gain medium and absorber saturation dynamics, leading to a dependence of the pulse duration and repetition rate on pump power. This is experimentally observed by changing the pump power. As shown in Fig. 5(d), the intracavity single pulse energy is varied from 6 nJ (corresponding to the threshold of -switching and below this the laser generates a continuous wave) to 9.5 nJ and the corresponding pulse duration is reduced from 6.6 μs to 4 μs while the cavity repetition rate is increased from 25.2 kHz to 50.7 kHz.
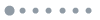
Figure 5.Typical output characteristics of the -switched pulses at a pump power of 27.5 mW: (a) optical spectrum; (b) single pulse profile, with a 4.49 μs FWHM pulse width; and (c) radio frequency spectrum of fundamental frequency on a 10 kHz span, where . (d) Variation of the pulse duration and repetition rate with pump power for the -switched Er-doped fiber laser using the few-layer SA.
Following stable mode-locked and -switched operation results achieved by using the microfiber-based SA device in the fiber laser cavity, the same experiments were conducted using the same microfiber after removing the deposited . Neither mode-locking nor -switching could be observed at any power level or polarization controller position, confirming that the saturable absorption behavior is attributed to the nonlinear optical response of at .
3. CONCLUSION
In summary, we have demonstrated the strong saturable absorption of a microfiber-based SA device fabricated by using an optical deposition method. This device is used to achieve self-starting mode-locking and -switching operation in an Er-doped fiber laser. Our work highlights the potential of -based SA devices as a promising ultrafast optical switch for versatile photonic applications, including ultrashort pulse generation.
References
[1] R. I. Woodward, E. J. R. Kelleher. 2D saturable absorbers for fibre lasers. Appl. Sci., 5, 1440-1456(2015).
[2] M. I. Dzhibladze, Z. G. Esiashvili, E. S. Teplitskii, S. K. Isaev, V. R. Sagaradze. Mode locking in a fiber laser. Sov. J. Quantum Electron., 13, 245-247(1983).
[3] U. Keller. Recent developments in compact ultrafast lasers. Nature, 424, 831-838(2003).
[4] T. Skaltsas, S. Pispas, N. Tagmatarchis. Photoinduced charge-transfer interactions on a graphene/block copolymer electrostatically bound to tetracationic porphyrin in aqueous media. Chem. Eur. J., 19, 9286-9290(2013).
[5] M. Zhang, E. J. R. Kelleher, F. Torrisi, Z. Sun, T. Hasan, D. Popa, F. Wang, A. C. Ferrari, S. V. Popov, J. R. Taylor. Tm-doped fiber laser mode-locked by graphene-polymer composite. Opt. Express, 20, 25077-25084(2012).
[6] M. Zhang, R. C. T. Howe, R. I. Woodward, E. J. R. Kelleher, F. Torrisi, G. Hu, S. V. Popov, J. R. Taylor, T. Hasan. Solution processed MoS2-PVA composite for sub-bandgap mode-locking of a wideband tunable ultrafast Er:fiber laser. Nano Res., 8, 1522-1534(2015).
[7] C. Liao, K. Fan, R. Xu, H. Zhang, C. Lu, Y. Cui, J. Zhang. Laser-annealing-made amplified spontaneous emission of ‘giant’ CdSe/CdS core/shell nanocrystals transferred from bulk-like shell to quantum-confined core. Photon. Res., 3, 200-205(2015).
[8] D. Mao, Y. Wang, C. Ma, B. Jiang, X. Gan, S. Hua, W. Zhang, T. Mei, J. Zhao. WS2 mode-locked ultrafast fiber laser. Sci. Rep., 5, 7965(2015).
[9] Z. Luo, D. Wu, B. Xu, H. Xu, Z. Cai, J. Peng, J. Weng, S. Xu, C. Zhu, F. Wang, Z. Sun, H. Zhang. Two-dimensional material-based saturable absorbers: towards compact visible-wavelength all-fiber pulsed lasers. Nanoscale, 8, 1066-1072(2016).
[10] G. Hu, T. Albrow-Owen, X. Jin, A. Ali, Y. Hu, R. C. T. Howe, K. Shehzad, Z. Yang, X. Zhu, R. I. Woodward, T.-C. Wu, H. Jussila, J.-B. Wu, P. Peng, P.-H. Tan, Z. Sun, E. J. R. Kelleher, M. Zhang, Y. Xu, T. Hasan. Black phosphorus ink formulation for inkjet printing of optoelectronics and photonics. Nat. Commun., 8, 278(2017).
[11] J. Du, M. Zhang, Z. Guo, J. Chen, X. Zhu, G. Hu, P. Peng, Z. Zheng, H. Zhang. Phosphorene quantum dot saturable absorbers for ultrafast fiber lasers. Sci. Rep., 7, 42357(2017).
[12] Y. Xu, X.-F. Jiang, Y. Ge, Z. Guo, Z. Zeng, Q.-H. Xu, H. Zhang, X.-F. Yu, D. Fan. Size-dependent nonlinear optical properties of black phosphorus nanosheets and their applications in ultrafast photonics. J. Mater. Chem. C, 5, 3007-3013(2017).
[13] J. Li, H. Luo, B. Zhai, R. Lu, Z. Guo, H. Zhang, Y. Liu. Black phosphorus: a two-dimension saturable absorption material for mid-infrared Q-switched and mode-locked fiber lasers. Sci. Rep., 6, 30361(2016).
[14] M. Zhang, G. Hu, G. Hu, R. C. T. Howe, L. Chen, Z. Zheng, T. Hasan. Yb- and Er-doped fiber laser Q-switched with an optically uniform, broadband WS2 saturable absorber. Sci. Rep., 5, 17482(2015).
[15] M. Barawi, E. Flores, M. Ponthieu, J. Ramón Ares, F. Cuevas, F. Leardini, I. Ferrer, C. Sanchez. Hydrogen storage by titanium based sulfides: nanoribbons (TiS3) and nanoplates (TiS2). J. Electr. Eng., 3, 24-29(2015).
[16] K. Dolui, S. Sanvito. Dimensionality-driven phonon softening and incipient charge density wave instability in TiS2. Europhys. Lett., 115, 47001(2016).
[17] S. Lin, Y. Chui, Y. Li, S. P. Lau. Liquid-phase exfoliation of black phosphorus and its applications. FlatChem, 2, 15-37(2017).
[18] Z. Guo, H. Zhang, S. Lu, Z. Wang, S. Tang, J. Shao, Z. Sun, H. Xie, H. Wang, X.-F. Yu, P. K. Chu. From black phosphorus to phosphorene: basic solvent exfoliation, evolution of Raman scattering, and applications to ultrafast photonics. Adv. Funct. Mater., 25, 6996-7002(2015).
[19] , S. Jiménez, X. K. Chen, J. C. Irwin. Raman spectra of Agx TiS2, and lattice dynamics of TiS2. Phys. Rev. B, 45, 14347-14353(1992).
[20] M. Scharli, F. Lévy. Interlayer acoustic phonons in the layered compound TiS2. Phys. Rev. B, 33, 4317-4319(1986).
[21] Z. Wang, L. Zhan, J. Wu, Z. Zou, L. Zhang, K. Qian, L. He, X. Fang. Self-starting ultrafast fiber lasers mode-locked with alcohol. Opt. Lett., 40, 3699-3702(2015).
[22] E. Garmire. Resonant optical nonlinearities in semiconductors. IEEE J. Sel. Top. Quantum Electron., 6, 1094-1110(2000).
[23] D. U. Noske, N. Pandit, J. R. Taylor. Source of spectral and temporal instability in soliton fiber lasers. Opt. Lett., 17, 1515-1517(1992).
[24] H. Zhang, D. Y. Tang, L. M. Zhao, N. Xiang. Coherent energy exchange between components of a vector soliton in fiber lasers. Opt. Express, 16, 12618-12623(2008).
[25] D. Y. Tang, H. Zhang, L. M. Zhao, X. Wu. Observation of high-order polarization-locked vector solitons in a fiber laser. Phys. Rev. Lett., 101, 153904(2008).
[26] R. I. Woodward, R. C. T. Howe, T. H. Runcorn, G. Hu, F. Torrisi, E. J. R. Kelleher, T. Hasan. Wideband saturable absorption in few-layer molybdenum diselenide (MoSe2) for Q-switching Yb-, Er- and Tm-doped fiber lasers. Opt. Express, 23, 20051-20061(2015).
[27] Y. Z. Huang, Z. Q. Luo, Y. Y. Li, M. Zhong, B. Xu, K. J. Che, H. Y. Xu, Z. P. Cai, J. Peng, J. Weng. Widely-tunable, passively Q-switched erbium-doped fiber laser with few-layer MoS2 saturable absorber. Opt. Express, 22, 25258-25266(2014).
[28] P. G. Ge, J. Liu, S. Z. Jiang, Y. Y. Xu, B. Y. Man. Compact Q-switched 2 μm Tm:GdVO4 laser with MoS2 absorber. Photon. Res., 3, 256-259(2015).
[29] Z. Q. Luo, Y. Z. Huang, M. Zhong, Y. Y. Li, J. Y. Wu, B. Xu, H. Y. Xu, Z. P. Cai, J. Peng, J. Weng. 1-, 1.5-, and 2-μm fiber lasers Q-switched by a broadband few-layer MoS2 saturable absorber. J. Lightwave Technol., 32, 4679-4686(2014).